Winemaking Affects Aquatic Ecosystem
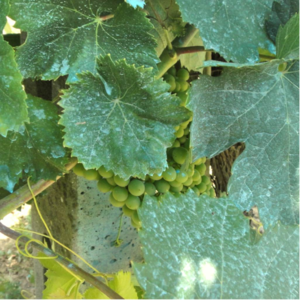
Winemaking Process and Runoff
In vinification, the grapes are first grown and harvested. Grape juices are then fermented and treated. The quality of wine is heavily dependent on the grapes used.[2]
In order to produce high quality wine while lowering production costs, vintners need to find economic methods to aid grape growth. Fungus is a common cause of disease in grape species, and can lead to severe economic loss.[3]
To protect grapevines against fungal infections, winemakers use a fungicide called Bordeaux Mixture.[4]
The Bordeaux Mixture is composed of copper(II) sulfate (CuSO4), calcium hydroxide (Ca(OH)2), and water. This combination is highly effective and long-lasting. Bordeaux Mixture enables cupric ion-mediated inhibition of enzymes involved in fungal spore formation.[5]
However, the Bordeaux Mixture is also more phytotoxic than other fungicides.[6]
Due to the solubility of copper(II) sulfate, Bordeaux Mixture is susceptible to runoff.[7]
In 2009, the wine producing province of Champagne in France contained exceptionally high copper content in its water systems, with a mean copper content of 53.6 μg/dm3 in basin water samples collected locally, and 24% of samples reached over 100 μg/dm3 of copper content according to an ecological survey.[8]
This presents a severe issue to both the aquatic ecosystem and human health, as long-term exposure to copper contaminated foods can lead to chronic diseases.[9]
Recent studies and bioassays conducted have been focused on the threshold level and control of copper levels, and subsequent effect and recovery of aquatic ecosystems.
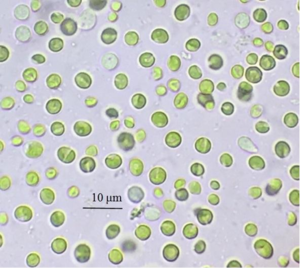
Use of Algae as Model Organisms
Algae such as Chlorella vulgaris are primarily discussed as a model organism. C. vulgaris is a single celled green spherical microalga found naturally in freshwater.[11] C. vulgaris and other algae are susceptible to minor changes to its immediate environment, therefore extensively used in studies in population dynamics and bioassays.[12] In addition to the sensitivity to environment, C. vulgaris can be easily grown and observed in a laboratory setting, making it a prime organism to model algae. Other aquatic organisms are also taken into account. Marine organisms, namely saltwater algae, usually display lower copper tolerance than freshwater algae, and will be affected by vineyard runoff,[13] but will not be discussed in detail. “Algae” in this page is used when a study examined multiple species of algae, and those species displayed a consistent response. Species names are used when a study only examined one species or that different species displayed different responses.
Effect of Varying Copper Concentrations
Trace amounts of copper ions have negligible effects on algal growth, and are not a main contributor to ecological damage.[14]
At concentrations of 10-15 to 10-7 M, the algal growth is enhanced due to natural demand for cupric ions as nutrients.[13]
At higher copper concentrations, below 1 mg/L, cupric ions have an adverse effect on algal growth.[15]
The relationship between copper concentration and algae growth rate is logarithmic, with a 40% decline in growth rate at 0.005 mg/L, 50% between 0.015 and 0.020 mg/L, and 80% at 0.1 mg/L of copper.[16]
As a coping mechanism against high concentrations of heavy metal, including copper, algae can regulate the amount of copper intake.[17]
The copper that was taken up can be immobilized intracellularly.[13]
At concentrations above 1 mg/L of copper, the growth of algae is greatly reduced, while between 2 and 5 mg/L of copper shows no difference in effects on C. vulgaris. The higher copper concentration also promotes a disruptive selection pattern in algal cell size, namely large and small cells are favored over average-sized cells.[18]
The LD50 critical concentration of copper is 5 mg/L.[19]
The tolerance of algae for copper is decreased when compounded with other metal toxicants such as silver ions. In the presence of 0.75 mg/L of colloidal silver ions, 0.2 mg/L of copper is effective in controlling algal population.[20]
Overall, the phytotoxicity of copper is likely compounded by the presence of other metal ions.[21]
Mechanism of Inhibition
In the presence of cupric ions, copper can associate with organic molecules, forming metal-organic frameworks (MOFs), and cause oxidative stress to the cell.[22]
The dissociation of MOFs can introduce excessive cupric ions into the cell.[23]
Intracellular cupric ions inhibit enzymes, resulting in decreased cell metabolism.[24]
Physical binding of cupric ions onto the cell surface is also a mechanism of damage. Incorporation of cupric ions into the cell membrane can cause mechanical damage, and the positive charge of cupric ions can also induce oxidative damage. Such binding can also negatively impact the efficiency of nutrient uptake and reduce sunlight availability, thus inhibiting the growth of algae.[25]
Effects on Aquatic Ecosystem
Aquatic micro-animals such as rotifers are more tolerant to copper concentrations compared to algae. However, rotifers are still severely affected after being exposed to 0.5 mg/L copper treatment for a week.[16]
Studies done on juvenile rainbow trout show that copper accumulates in the gills and intestines and decreases Na+/K+-ATPase activity in the gills and intestines. However, although no statistically significant copper accumulates in the spleen or brain, Na+/K+-ATPase activity also decreases in the brain, which suggests that copper in gills may contribute to hypoxia.[26]
Other effects of vineyard copper runoff on fish are identified using zebrafish as a model organism. On a physiological and behavioral level, zebrafish exposed to vineyard watershed has an elevated level of cortisol and spends more time in deeper water than shallow water.[7]
The immediate effect of copper on the overall ecosystem includes decrease in algal population and depletion of dissolved oxygen. The short-term effect includes accelerated phosphorus recycling coupled with recovery of algal population and sporadic killing of fish. Long term effects include copper accumulation in sediments, adaptation of algae for higher copper tolerance, abundance shift from game fish to rough fish and green algae to blue-green algae, killing of macrophytes, and reduced population in benthic invertebrates.[27]
Bioremediation as a Potential Method for Restoration
Researches into effect of copper on various organisms have given potential methods for lowering copper content in waters currently affected by copper runoff. One such method is bioremediation using algae. Sargassum, a macroalga, has a higher rate of copper removal with a maximum copper uptake of 71.4 mg/g, followed by 19.3 mg/g for Chlorococcum, a microalga, and 11.4 mg/g for activated carbon.[28] However, Sargassum is a saltwater alga. Although Sargassum possesses the highest copper uptake by mass, it is ineffective in freshwater. Spirulina platensis is a cyanobacterium that can also be used as a biofilter in freshwater, with a maximum biosorption of 90.6% of copper ions in medium containing 100 mg/L of copper at 37 °C in 90 minutes with 0.050 g of dry biomass.[29] The use of Spirulina offers other benefits, as Spirulina can be harvested and used as biofuel, a cleaner alternative to traditional fossil fuels.[30] Spirulina can also be converted into fertilizers, and the high copper content of cells used for biofiltration can provide a source of copper for agriculture.[31] This provides unique opportunities in the use of Spirulina and similar algae in bioremediation of vineyard aquatic ecosystems.
References
- ↑ Lorthiois, G., 2020. Bordeaux mixture, an effective treatment. [Online] Available at: https://www.nature-and-garden.com/gardening/bordeaux-mixture.html [Accessed 2 December 2021].
- ↑ Amerine, M. A., 2021. Wine. [Online] Available at: https://www.britannica.com/topic/wine [Accessed 20 November 2021].
- ↑ University of Maryland, 2021. Grape Diseases. [Online] Available at: https://extension.umd.edu/resource/grape-diseases [Accessed 20 November 2021].
- ↑ Schilder, A., 2010. Fungicide properties and weather conditions. [Online] Available at: https://www.canr.msu.edu/news/fungicide_properties_and_weather_conditions [Accessed 11 November 2021].
- ↑ Liu, H. et al., 2015. Copper Ion Elicits Defense Response in Arabidopsis thaliana by Activating Salicylate- and Ethylene-Dependent Signaling Pathways. Molecular Plant, 8(10), pp. 1550-1553.
- ↑ Broome, J. C. & Donaldson, D. R., 2010. Bordeaux Mixture. [Online] Available at: http://ipm.ucanr.edu/PMG/PESTNOTES/pn7481.html [Accessed 20 November 2021].
- ↑ 7.0 7.1 Pompermaier, A. et al., 2021. Water and suspended sediment runoff from vineyard watersheds affecting the behavior and physiology of zebrafish. Science of The Total Environment, 757(1).
- ↑ Banas, D. et al., 2010. Copper mobilization affected by weather conditions in a stormwater detention system receiving runoff waters from vineyard soils (Champagne, France). Environmental Pollution, 158(2), pp. 476-482.
- ↑ European Food Safety Authority, 2021. Metals as contaminants in food. [Online] Available at: https://www.efsa.europa.eu/en/topics/topic/metals-contaminants-food [Accessed 25 February 2021].
- ↑ Ramaraj, R., Unpaprom, Y. & Dussadee, N., 2016. Cultivation of Green Microalga, Chlorella vulgaris for Biogas Purification. International Journal of Scientific & Technology Research, 2(3), pp. 117-122.
- ↑ Universal Protein Resource, 2018. Taxonomy - Chlorella vulgaris (Green alga). [Online] Available at: https://www.uniprot.org/taxonomy/3077 [Accessed 9 May 2020].
- ↑ Bi, R. et al., 2018. Sensitivities of seven algal species to triclosan, fluoxetine and their mixtures. Scientific Reports, s.l.(s.l.), p. s.l..
- ↑ 13.0 13.1 13.2 Knauer, K., Behra, R. & Sigg, L., 2009. Effects of free Cu2+ and Zn2+ ions on growth and metal accumulation in freshwater algae. Environmental Toxicology, 16(2), pp. 220-229.
- ↑ Tubbing, D. M. et al., 1994. The contribution of complexed copper to the metabolic inhibition of algae and bacteria in synthetic media and river water. Water Research, 28(1), pp. 37-44.
- ↑ Moss, V. A., Roberts, J. A., Keith, H. & Simpson, L., 1977. The effect of copper sulfate on the growth of the alga Chlorella. British Homeopathic Journal, 66(3), pp. 169-177.
- ↑ 16.0 16.1 Brian, T. K. & Meadows, S., 1978. The toxic effect of copper on algae and rotifers from a Soda Lake (Lake Nakuru, East Africa). Water Research, 12(10), pp. 771-775.
- ↑ Trollope, D. R. & Evans, B., 1976. Concentrations of copper, iron, lead, nickel and zinc in freshwater algal blooms. Environmental Pollution (1970), 11(2), pp. 109-116.
- ↑ Liu, S., 2021. What are the recovery rates of C. vulgaris populations exposed to copper(II) sulfate (CuSO4) at different concentrations (0.0ppm, 0.2ppm, 0.4ppm, 0.6ppm, 0.8ppm, and 1.0ppm) and different visible growing light colors (White, Red, Blue, Green) over 7 days (168 hours)?. Beijing World Youth Academy. Unpublished.
- ↑ Fan, G., Zhou, J., Zheng, X. & Chen, W., 2018. Growth Inhibition of Microcystis aeruginosa by Copper-based MOFs: Performance and Physiological Effect on Algal Cells. Applied Organometallic Chemistry, 32(12).
- ↑ Fitzgerald, G. P., Gerloff, G. C. & Skoog, F., 1952. Studies on Chemicals with Selective Toxicity to Blue-Green Algae. Sewage and Industrial Wastes, 24(7), pp. 888-896.
- ↑ Young, R. G. & Lisk, D. J., 1972. Effect of Copper and Silver Ions on Algae. Journal (Water Pollution Control Federation), 44(8), pp. 1743-1647.
- ↑ Fan, G. et al., 2019. Growth inhibition of harmful cyanobacteria by nanocrystalline Cu-MOF-74: Efficiency and its mechanisms. Journal of Hazardous Materials, 367(1), pp. 529-538.
- ↑ Li, Y., Shang, S., Shang, J. & Wang, W.-X., 2021. Toxicity assessment and underlying mechanisms of multiple metal organic frameworks using the green algae Chlamydomonas reinhardtii model. Environmental Pollution, 291(1).
- ↑ Australian Government, 1996. Toxicity assessment of waters from Macquarie Harbour, western Tasmania, using algae, invertebrates and fish, Canberra: Department of Agriculture, Water and the Environment.
- ↑ Zhu, X. et al., 2020. Growth inhibition of the microalgae Skeletonema costatum under copper nanoparticles with microplastic exposure. Marine Environmental Research, 158(1).
- ↑ Shaw, B. J., Al-Bairuty, G. & Handy, R. D., 2012. Effects of waterborne copper nanoparticles and copper sulfate on rainbow trout, (Oncorhynchus mykiss): Physiology and accumulation. Aquatic Toxicology, 116-117(1), pp. 90-101.
- ↑ Hanson, M. J. & Stefan, H. G., 1984. Side Effects of 58 Years of Copper Sulfate Treatment of the Fairmont Lakes, Minnesota. Journal of the American Water Resources Association, 20(6), pp. 889-900.
- ↑ Jacinto, M. L. J., David, C. P. C., Perez, T. R. & Jesus, B. R. D., 2009. Comparative efficiency of algal biofilters in the removal of chromium and copper from wastewater. Ecological Engineering, 35(5), pp. 856-860.
- ↑ Al-Homaidan, A. A. et al., 2014. Biosorption of copper ions from aqueous solutions by Spirulina platensis biomass. Arabian Journal of Chemistry, 7(1), pp. 57-62.
- ↑ Rahman, M. et al., 2017. Biodiesel production from microalgae Spirulina maxima by two step process: Optimization of process variable. Journal of Radiation Research and Applied Sciences, 10(2), pp. 140-147.
- ↑ Alobwede, E., Leake, J. R. & Pandhal, J., 2019. Circular economy fertilization: Testing micro and macro algal species as soil improvers and nutrient sources for crop production in greenhouse and field conditions. Geoderma, 334(1), pp. 113-123.
Edited by Sizhuo Liu, student of Joan Slonczewski for BIOL 116 Information in Living Systems, 2021, Kenyon College.