Rhodopseudomonas palustris and extracellular electron transfer: Difference between revisions
No edit summary |
|||
(19 intermediate revisions by one other user not shown) | |||
Line 1: | Line 1: | ||
{{Uncurated}} | |||
==Introduction== | ==Introduction== | ||
[[Image:Figure 1 Rhodopseudomonas Palustris Cinti.jpg|thumb|300px|right|Figure 1. Colorized Scanning Electron Micrograph of Rhodopseudomonas Palustris. ) http://biotechdesk.com/weekly-articles/microbes-live-on-electricity/attachment/f1-medium]] | [[Image:Figure 1 Rhodopseudomonas Palustris Cinti.jpg|thumb|300px|right|Figure 1. Colorized Scanning Electron Micrograph of Rhodopseudomonas Palustris. ) http://biotechdesk.com/weekly-articles/microbes-live-on-electricity/attachment/f1-medium]] | ||
Line 19: | Line 20: | ||
<b> The PioABC operon: </b> | <b> The PioABC operon: </b> | ||
These findings were insightful because it revealed that <i>R. palustris</i> was capable of taking up electrons from solid-phase materials if it was able to take up electrons from the current generated by an electrode. Ferrous iron was not necessary for the bacterium as the researchers previously thought (1). To examine which genetic component was at work here, the researchers turned to the gene sequence that the bacterium uses when it obtain electrons from Fe(II). They knew that the proteins involved in the electron transfer from Fe(II) to the electron transport system are coded by the pioABC operon (1). The pioABC system, it turns out, also affects the electron uptake that was observed from the electrode. By inoculating strains of <i>R. palustris</i> to render them pioABC deficient (ΔpioABC) and then comparing these strains with the wild-type strains, they found that the mutant accepted 30% less current from the electrode than the wild-type strain in the light treatments. In addition, expression of certain pioABC proteins was upregulated in the wild-type strain during electron uptake. Protein expression of the pioABC was measured by its corresponding mRNA abundance. The researchers found that the pioA protein was upregulated the most in biofilms of <i>R. palustris</i> in illuminated conditions. pioA is a periplasmic decaheme cytochrome that is one of the key proteins responsible for ATP generation during electron transport (8), and so it is intuitive that this protein was regulated the most in the presence of light (Figure 5). This level of expression was comparable to the expression of pioC observed under photoferrotrophic growth on Fe(II) in a conventional culture apparatus. PioC is a periplasmic high potential iron-sulfur cluster protein. | These findings were insightful because it revealed that <i>R. palustris</i> was capable of taking up electrons from solid-phase materials if it was able to take up electrons from the current generated by an electrode. Ferrous iron was not necessary for the bacterium as the researchers previously thought (1). To examine which genetic component was at work here, the researchers turned to the gene sequence that the bacterium uses when it obtain electrons from Fe(II). They knew that the proteins involved in the electron transfer from Fe(II) to the electron transport system are coded by the pioABC operon (1). The pioABC system, it turns out, also affects the electron uptake that was observed from the electrode. By inoculating strains of <i>R. palustris</i> to render them pioABC deficient (ΔpioABC) and then comparing these strains with the wild-type strains, they found that the mutant accepted 30% less current from the electrode than the wild-type strain in the light treatments. In addition, expression of certain pioABC proteins was upregulated in the wild-type strain during electron uptake. Protein expression of the pioABC was measured by its corresponding mRNA abundance. The researchers found that the pioA protein was upregulated the most in biofilms of <i>R. palustris</i> in illuminated conditions. pioA is a periplasmic decaheme cytochrome that is one of the key proteins responsible for ATP generation during electron transport (8), and so it is intuitive that this protein was regulated the most in the presence of light (Figure 5). This level of expression was comparable to the expression of pioC observed under photoferrotrophic growth on Fe(II) in a conventional culture apparatus. PioC is a periplasmic high potential iron-sulfur cluster protein. pioC expression was highest in the photoferrotrophic growth because the presence of iron stiumulated pioC to perform oxidation and reduction reactions. Expression of pioA was relatively low under these conditions, further suggesting that pioA is most active with the presence of light. Planktonic cells of <i>R. palustris</i>, which refer to cells that did not form biofilms on the electrode, exhibited lower levels of pio protein expression in illuminated conditions. As expected, the inoculum strain also exhibited very little expression. Together, this data suggests that the pioABC operon is one of the key molecular mechanisms responsible for extracellular electron transfer within <i>R. palustris</i>. It also indicates that the expression of certain proteins are favored when the bacteria has formed biofilms on the electrode. Biofilms and their relationship to extracellular transfer is addressed later on this page. | ||
[[Image:Figure 6 mRNA expression pioABC Cinti.jpeg |thumb|300px|right|Figure 5: Current uptake and mRNA abundance in the pioABC operon under various growth conditions.(Bose, A., Gardel, E.J., Girguis, P.R., Parra, E.A., Vidoudez, C., Electron uptake by iron-oxidizing phototrophic bacteria, Nature Communications, 2014, DOI: 10.1038/ncomms4391).]] | [[Image:Figure 6 mRNA expression pioABC Cinti.jpeg |thumb|300px|right|Figure 5: Current uptake and mRNA abundance in the pioABC operon under various growth conditions.(Bose, A., Gardel, E.J., Girguis, P.R., Parra, E.A., Vidoudez, C., Electron uptake by iron-oxidizing phototrophic bacteria, Nature Communications, 2014, DOI: 10.1038/ncomms4391).]] | ||
<b> Expression of ruBisCO: </b> | <b> Expression of ruBisCO: </b> | ||
The researches utilized transcriptome analysis to sequence RNA strains of <i>R. palustris</i> to examine the role of the enzyme ruBisCO in electron uptake. RuBisCO is essential to all photosynthetic organisms because it is responsible for the conversion of carbon dioxide to energy-rich molecules like glucose. It does this by catalyzing the carboxylation of ribulose-1,5-bisphosphate, yielding two molecules of 3-phosphoglycerate which can then be reduced by NADPH in the Calvin cycle. <i>R. palustris</i> is able to express two different forms of ruBisCO, noted as Form I and II. | The researches utilized transcriptome analysis to sequence RNA strains of <i>R. palustris</i> to examine the role of the enzyme ruBisCO in electron uptake. RuBisCO is essential to all photosynthetic organisms because it is responsible for the conversion of carbon dioxide to energy-rich molecules like glucose (9). It does this by catalyzing the carboxylation of ribulose-1,5-bisphosphate, yielding two molecules of 3-phosphoglycerate which can then be reduced by NADPH in the Calvin cycle (1). <i>R. palustris</i> is able to express two different forms of ruBisCO, noted as Form I and II. Both forms perform similar functions and are responsible for the fixation of carbon dioxide, yet Girguis et al. found that form I was more highly expressed in the wild-type strain in the light treatments than form II (1). Also, expression of ruBisCO form I was significantly lower in the dark treatments. It has been known that in the presence of light, microbes absorb light and as a result produce ATP and NADPH. These energy carriers have been shown to positively regulate ruBisCO expression (9). In the dark, much less light can be absorbed by the microbe and so these energy-rich metabolites are much less abundant. If ATP and NADPH are low in abundance, ruBisCO expression will decrease. | ||
<i>R. palustris</i> purposefully remains towards the surface of soil and water | <i>R. palustris</i> purposefully remains towards the surface of soil and water so that it can as close to sunlight as possible. This way, ruBisCO can be activated more regularly and electron uptake can occur. Yet the researches found that much of the ferrous minerals that it acquires electrons from lie deep within sediment because they are insoluble (1). In this way, <i>R. palustris</i> is unique among bacteria in its ability to access energy from both underground minerals and sunlight. <br> | ||
==Nitrogen fixation and Hydrogen Production== | ==Nitrogen fixation and Hydrogen Production== | ||
[[Image:Figure_5_Vanadium_nitrogense_Cinti.jpeg|thumb|300px|left|Figure 6: Vanadium Nitrogenase, found in members of the beacteria genus Azotobacter as well as the species Rhodopseudomonas Palustris.http://en.wikipedia.org/wiki/Vanadium_nitrogenase]] | [[Image:Figure_5_Vanadium_nitrogense_Cinti.jpeg|thumb|300px|left|Figure 6: Vanadium Nitrogenase, found in members of the beacteria genus Azotobacter as well as the species Rhodopseudomonas Palustris.http://en.wikipedia.org/wiki/Vanadium_nitrogenase]] | ||
Another important aspect of <i>R. palustris's</i> metabolism is its highly efficient way of producing hydrogen. Hydrogen is a particularly efficient fuel source because it can be easily combined with oxygen to produce electricity, heat, and water. Many issues arise with using hydrogen as a primary fuel source to power automobiles and homes, one of them being that unless it is in liquid form, which requires energy to maintain, hydrogen gas takes up too much volume and can be hard to store (10). Nevertheless, hydrogen is produced as a byproduct of nitrogen fixation in many bacteria. Nitrogen-fixing bacteria use nitrogenases to convert nitrogen gas into ammonia, a usable form of nitrogen to plants (7). Nitrogenases also reduce protons to hydrogen gas (7). <i>R. palustris</i> is able to produce nearly three times as much hydrogen as most other nitrogen-fixing bacteria because it codes for an enzyme called vanadium nitrogenase (Figure 6). Vanadium nitrogenase is found in members of the Azotobacter but is also found in the species <i>R. palustris</i> and <i>Anabaena variabilis</i> (7). This differs from the more common nitrogen-fixing enzyme, molybdenum nitrogenase, in its ability to reduce more protons and thus generate more hydrogen gas. Along with cyanobacteria, <i>R. palustris</i> have been incorporated in microreactors to produce hydrogen that can be used for practical uses (5). | |||
==Electrical conductivity of biofilms== | ==Electrical conductivity of biofilms== | ||
The formation of biofilms can play an important role in extracellular electron transfer. A biofilm is when a group of microorganisms stick to each other on a surface. Biofilms form for a number of reasons, but the primary reason microbes form biofilms is in response to a lack of nutrients (11). Biofilms also have unique properties with heat and electricity. Previously, it was known that biofilms could only withhold insulation (2). But a recent study done by Malvankar et al. (2012) on <i>Geobacter sulfurreducens</i> shows shows that both mixed-species and pure culture biofilms have the potential to conduct electricity. This is an important finding because it revealed that upon electron uptake, bacterium in a biofilm that were not in direct contact with an electrode contributed to current production equally as much as bacterium in direct contact. Thus, all bacterium in a biofilm have the potential to employ extracellular electron transfer, no matter how close they are to the direct source (2). This could potentially help explain why <i> R. palustris </i> was capable of extracting electrons from material deep within sediment. | |||
==Flavins and Quinones Enhance EET== | ==Flavins and Quinones Enhance EET== | ||
In a study conducted by Akihiro Okamato et. al in 2012, researchers examined the role of secreted flavins in <i>Shewanella oneidensis</i>'s metabolic processes. <i>S. oneidensis</i> is a gram-negative, anaerobically respiring bacterium that is often found in the deep-sea as well as soil and sedentary habitats (12). It is a member of the proteobacteria family and its genome is compacted within a single chromosome (12). <i>S. oneidensis</i> is another bacterium capable of extracellular electron transfer and it can use Fe(III) and Mn(IV)-oxide as its solid electron acceptors. The researchers were interested in how secreted flavins influence the metal reduction pathway, which is responsible for metal-oxide reduction reactions and the production of anodic current from the inner cellular membrane to the outer cellular membrane (3). Previous studies have showed that certain flavins, such as riboflavin and flavin mononucleotide, facilitate the metal reduction pathway by interacting with the OM protein MtrC (3). MtrC is a a 71-kDa decaheme c-Cytochrome that is responsible for catalyzing reactions within the metal reduction pathway and it is found on the outer membrane. Flavins are capable of increasing MtrC enzymatic activity by accessing heme centers on the exterior or buried within the protein scaffolds where they can serve as primary electron acceptors for the reduced hemes (3). Okamato et. al sought out to to further understand the mechanism by which flavins enhance the metal reduction pathway. | |||
[[Image:Figure_7_Cinti.jpeg |thumb|300px|right|Figure 7: DP voltammograms of monolayer biofilms of <i>S. oneidensis</i> with increasing increments of flavin. (Hashimoto, K., Nakamura, R., Nealson, K., Okamoto, A., Rate enhancement of bacterial extracellular electron transport involves bound flavin semiquinones, PNAS, 2012, DOI: 10.1073/pnas.1220823110.]] | [[Image:Figure_7_Cinti.jpeg |thumb|300px|right|Figure 7: DP voltammograms of monolayer biofilms of <i>S. oneidensis</i> with increasing increments of flavin. (Hashimoto, K., Nakamura, R., Nealson, K., Okamoto, A., Rate enhancement of bacterial extracellular electron transport involves bound flavin semiquinones, PNAS, 2012, DOI: 10.1073/pnas.1220823110.]] | ||
Similar to the study done by Girguis et. al, the researchers fabricated a monolayer biofilm of <i>S. oneidensis</i> on flat indium tin-oxide electrodes and used voltammetric techniques to monitor the redox properties of this species. Monolayer biofilms were exposed to increasing concentrations of flavin, and as expected, increased flavin concentration resulted in higher redox potentials within <i>S. oneidensis</i> cells (Figure 7). The researchers then studied flavin's interaction with the MtrC protein. It is important to note that when flavin (FMN) is fully oxidized, it can be reduced in either a one-electron reaction to form a derivative called semiquinone (sq), or a two-electron reaction to form hydroquinone (hq) (3). Semiquinone presence was measured in mutant strains of <i>S. oneidensis</i> lacking the ability to produce MtrC proteins (ΔmtrC) and OmcA proteins (ΔomcA). The ΔmtrC strain showed low levels of semiquinone formation, while the lack of OmcA proteins provided little effect on the formation of semiquinone. Taken together, these results suggest that the semiquinone formed in this reduction reaction is stabilized by the specific interaction with the MtrC protein (3). | |||
[[Image:Figure_8_Cinti.jpeg|thumb|300px|left|Figure 8: (A) Current generation for <i>S. oneidensis</i> cells with the addition of lactate indicated. (B) before lactate addition and (C) after. (Hashimoto, K., Nakamura, R., Nealson, K., Okamoto, A., Rate enhancement of bacterial extracellular electron transport involves bound flavin semiquinones, PNAS, 2012, DOI: 10.1073/pnas.1220823110.]] | [[Image:Figure_8_Cinti.jpeg|thumb|300px|left|Figure 8: (A) Current generation for <i>S. oneidensis</i> cells with the addition of lactate indicated. (B) before lactate addition and (C) after. (Hashimoto, K., Nakamura, R., Nealson, K., Okamoto, A., Rate enhancement of bacterial extracellular electron transport involves bound flavin semiquinones, PNAS, 2012, DOI: 10.1073/pnas.1220823110.]] | ||
Semiquinone formation was also found to be highly coupled to <i>S. oneidensis</i>'s metabolic activity. When grown with lactate as their main food source, cells are able to oxidize lactate and transfer the electrons to extracellular acceptors via the metal reduction pathway (3). Ultimately, the oxidation state of hemes in the protein reflect the balance between the electron input from respiration and the output due to extracellular electron transfer. Metabolically active cells require that most of the hemes in the MtrC protein are in a reduced state. In the absence of lactate, microbial current remained low. But when lactate was added to the culture, current increased (Figure 8) and the number of electrons of flavin reduction changed from 2 to 1. These findings allowed the researchers two make two very important conclusions. Microbial metabolism, which facilitates a continuous flow of electrons, is essential for this species of bacteria to use semiquinone as an enhancer of extracellular electron transfer. The other conclusion is that the activation of flavin is coupled to the nature of the oxidation state of the hemes in MtrC (3). | |||
==Various extracellular electron acceptors== | ==Various extracellular electron acceptors== | ||
From these two studies that addressed extracellular electron transfer, it is evident that the mechanisms behind this energy acquiring process is unique to the microbe. <i>R. palustris </i> was capable of extracellular electron transfer with ferrous iron has it's electron acceptor, but could also acquire electrons without it. <i>S. oneidensis</i> is capable of using Fe(III) and Mn(IV)-oxide as its solid electron acceptors. The range of solid-phase electron acceptors that are possible for extracellular electron transfer is quite extensive but has yet to be accurately determined. Many sulfate-reducing bacteria that use lactate as the electron donor can use | From these two studies that addressed extracellular electron transfer, it is evident that the mechanisms behind this energy acquiring process is unique to the microbe. <i>R. palustris </i> was capable of extracellular electron transfer with ferrous iron has it's electron acceptor, but could also acquire electrons without it. <i>S. oneidensis</i> is capable of using Fe(III) and Mn(IV)-oxide as its solid electron acceptors. The range of solid-phase electron acceptors that are possible for extracellular electron transfer is quite extensive but has yet to be accurately determined. Many sulfate-reducing bacteria that use lactate as the electron donor can use hannebachite (CaSO3), gypsum (CaSO4), anglesite (PbSO4), all low-solubility crystallines (4). More research needs to be done on why certain solid-phase minerals serve as electron acceptors and why some cannot. | ||
==Conclusion== | ==Conclusion== | ||
An advance in our understanding of <i>R. palustris</i> and <i>S. oneidensis</i> and how they undergo extracellular electron transfer has profound implications. Microbes that can perform electron extracellular transfer confer an ecological advantage – although soluble electron donors and acceptors are ubiquitous, the ability to acquire electrons from solid-phase materials can be beneficial in environments that are nutrient poor and uninhabitable to more basic microbes. Girguis’s study gave light to the possibility of using <i>R. palustris</i> in batteries or other practical appliances, although they were unsure that the bacterium would be a very efficient fuel source. If genes responsible for extracellular electron transfer were spliced into animal genomes or human genomes, could we acquire energy from our environment? This is a profound question that researchers are beginning to grapple with and explore. | |||
==References== | ==References== | ||
1) Bose, A., Gardel, E.J., Girguis, P.R., Parra, E.A., Vidoudez, C., <i> Electron uptake by iron-oxidizing phototrophic bacteria</i>, Nature Communications, 2014, DOI: 10.1038/ncomms4391 <br> | |||
<br> 2) Franks, A., Lau, J., Lovley, D., Malvankar, N., Nevin, K., Tuominen, M., <i> Electrical Conductivity in a Mixed-Species Biofilm</i>, Applied and Envrionmental Microbiology, 2012, Vol. 78, no.16 5967-5971. <br> | <br> 2) Franks, A., Lau, J., Lovley, D., Malvankar, N., Nevin, K., Tuominen, M., <i> Electrical Conductivity in a Mixed-Species Biofilm</i>, Applied and Envrionmental Microbiology, 2012, Vol. 78, no.16 5967-5971. <br> | ||
<br> 3) Hashimoto, K., Nakamura, R., Nealson, K., Okamoto, A., Rate enhancement of bacterial extracellular electron transport involves bound flavin semiquinones, PNAS, 2012, DOI: 10.1073/pnas.1220823110.<br> | <br> 3) Hashimoto, K., Nakamura, R., Nealson, K., Okamoto, A., Rate enhancement of bacterial extracellular electron transport involves bound flavin semiquinones, PNAS, 2012, DOI: 10.1073/pnas.1220823110.<br> | ||
<br> 4) Karnachuk OV1, Kurochkina SY, Tuovinen OH, Growth of sulfate-reducing bacteria with solid-phase electron acceptors, Pub Med, 2002.<br> | <br> 4) Karnachuk OV1, Kurochkina SY, Tuovinen OH, Growth of sulfate-reducing bacteria with solid-phase electron acceptors, Pub Med, 2002.<br> | ||
<br> 5) http://en.wikipedia.org/wiki/Rhodopseudomonas_palustris <br> | <br> 5) Rhodopseudomonas palustris, http://en.wikipedia.org/wiki/Rhodopseudomonas_palustris, 2014 <br> | ||
<br> 6) http://microbewiki.kenyon.edu/index.php/Rhodopseudomonas_palustris<br> | <br> 6) Rhodopseudomonas palustris and its role in bioremediation of soil pollutants, http://microbewiki.kenyon.edu/index.php/Rhodopseudomonas_palustris, 2014 <br> | ||
<br> 7) http://en.wikipedia.org/wiki/Vanadium_nitrogenase<br> | <br> 7) Vanadium nitrogenase, http://en.wikipedia.org/wiki/Vanadium_nitrogenase, 2014 <br> | ||
<br> 8) http://en.wikipedia.org/wiki/Cytochrome | <br> 8) Cytochrome, http://en.wikipedia.org/wiki/Cytochrome, 2014 <br> | ||
<br> 9) RuBisCO, http://en.wikipedia.org/wiki/RuBisCO, 2014 <br> | |||
<br> 10) Hydrogen fuel, http://en.wikipedia.org/wiki/Hydrogen_fuel, 2014 <br> | |||
<br> 11) Biofilm, http://en.wikipedia.org/wiki/Biofilm, 2014 <br> | |||
<br> 12) Shewanella oneidensis, http://microbewiki.kenyon.edu/index.php/Shewanella_oneidensis, 2014 |
Latest revision as of 20:29, 29 September 2015
Introduction
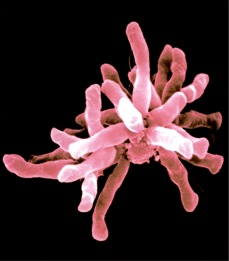
All life forms need energy to grow and survive. In order to grow, an organism needs energy to generate new cells from inorganic substances. For most organisms, this energy is derived from basic reduction-oxidation chemistry. Redox chemistry involves the transfer of electrons between two species, and this simple exchange is fundamental to processes like cellular respiration and photosynthesis. Photosynthesis occurs when carbon dioxide is reduced into sugars and water is oxidized to oxygen. Respiration, on the other hand, oxidizes sugars to yield carbon dioxide and water. Aside from these most basic processes, microbes are capable of yielding energy from virtually any chemical reaction that our biosphere can allow. To study the dynamics of these chemical reactions, microbiologists often turn to Rhodopseudomonas palustris. R.palustris is heavily studied because it is known by microbiologists as one of the most metabolically versatile bacterium on earth. R. palustris is a rod-shaped, gram-negative bacterium belonging to the proteobacteria family (6). It is typically found in soil, marine coast sediments and pond water (6). R. palustris have cells that are motile, exhibit polar growth, and reproduce via budding (Figure 1).
Metabolism
R. palustris falls under the category of purple non-sulfur bacteria. Purple non-sulfur bacteria are typically photohetorophic, meaning they absorb light without carbon dioxide fixation and acquire and assemble performed organic molecules from the outside. Yet R. palustris is unique to other purple non-sulfur bacteria in its ability to switch between photoheterotrophy, photoautotrophy, chemoautotrophy, and chemoheterotrophy (5) (Figure 2). The specific class of metabolism that the bacterium uses heavily depends on environmental conditions, such as the availability of light. When light is abundant, electrons enter the respiratory chain of donors and acceptors from a photo-excited chlorophyll, bacteriochlorophyl b (5). In addition to bacteriochlorophyl, R. palustris have developed intracytoplasmic membranes called thylakoids that help catalyze photosynthetic reactions under anaerobic conditions (5). In the presence of oxygen, the bacterium can degrade and accept electrons from performed organic molecules such as sugars and benzoates. R. palustris is also particularly adept at degrading sturdy, aromatic compounds and reducing them to usable substrates, eventually forming acetyl CoA and carbon dioxide (6). The acetyl CoA can then enter the triboxylic acid cycle (TCA) to produce energy for the organism. This bacterium has been of great use in removing environmental pollutants that contain benzene and cleaning up waste. Additionally, R. palustris can also utilize lithotrophy to acquire energy (5). This means it can accept electrons from inorganic compounds such as reduced sulfur and more prevalently, ferrous iron Fe(II) and channel them through the ETS to generate ATP.
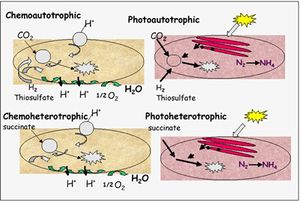
Extracellular electron transfer
Microbes typically require their electron donors and acceptors to be in solution. This way, the molecules donating or accepting the electrons can enter the cell of the microbe and be more readily available. Remarkably, a phylogenetically diverse branch of anaerobically respiring microbes can take electrons from external materials in the solid-phase (1). This process is known as extracellular electron transfer (EET). Little is known about EET and it is currently on the forefront of scientific research. Researchers are investing their time in understanding EET because it will allow us to better understand more practical microbe-mediated processes such as geochemical cycling, power generation in electrochemical cells, and bioremediation. These processes are essential to how we maintain our environment and how we distribute electrical power in this country. To dive deeper into extracellular electron transfer, R. palustris is a great model organism that has been identified as a bacterium capable of EET. A team led by Peter Girguis at Harvard recently examined R. palustris and its versatile metabolism to pinpoint the genetic determinants of EET and the molecular mechanisms that underlie it.
Bioelectrochemical systems to stimulate electron transfer:
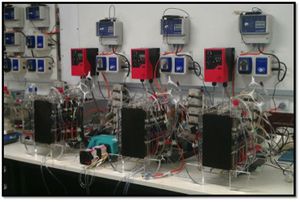
In this experiment conducted in July of 2013, Girguis et. al submerged electrodes in a bioreactor and allowed them to come into contact with R. palustris cells. The role of these bioelectrochemical systems (Figure 3) was to generate a current that mimicked the reduction potential of extracellular solid-phase minerals that R. palustris could use to metabolize (1). Using redox active minerals instead of an electrode posed many potential problems, one of them being the fear that during experimentation, changes in the composition of the minerals could occur. The researchers set up three different bioelectrochemical systems with differing conditions: In the first treatment, the bioreactor containing an electrode passing current was illuminated. In the second treatment, or the “dark treatment,” the bioreactor was non-illuminated. In the third treatment, also known as the control, illuminated bioreactors contained electrodes that had open circuits and therefore passed no current.
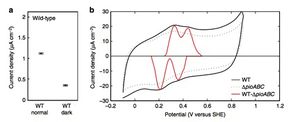
The researchers found that the bacterium exhibited the highest rate of electron uptake, measured in its current density, in the illuminated treatment (1)(Figure 4). The highest current served in this treatment was up to 1.5 mAcm^-2. Current uptake was detected in the dark treatments, yet the amount of current measured in the dark treatment was 70% lower than when illuminated. This puzzled the researchers because they had previously known that expression of ruBisCO, a key enzyme involved in carbon dioxide fixation, and the microbes ability to take up electrons are stimulated by light. Thus, they did not expect there to be any current exchange occurring in the dark treatments. They attributed this quandary to the fact that when light is unavailable, ATP cannot be synthesized through photosynthesis, yet cellular metabolism can continue as long as an electron donor is present. When light is available, however, R. palustrisis able to use photic energy to synthesize ATP from the electron transfer system without an electron donor. Electron donors are necessary only to produce reducing equivalents such as NADPH for cellular metabolism. Thus, the researchers believed that the observed current uptake in the dark treatment was simply showing the exchange of electrons from the electron donor. As expected, the electron uptake by R. palustris in the illuminated treatment was significantly higher than uptake in the dark treatment due to the fact the ATP generated in the light treatment is used for more energy-intensive cellular processes and therefore requires a higher level of electron uptake. The cells of R. palustris were exposed to the electrode for approximately 24 hours; longer exposure could add in additional factors that could affect experimental data such as changes in the bacterium's growth phases.
Genetic mechanisms involved in EET
The PioABC operon:
These findings were insightful because it revealed that R. palustris was capable of taking up electrons from solid-phase materials if it was able to take up electrons from the current generated by an electrode. Ferrous iron was not necessary for the bacterium as the researchers previously thought (1). To examine which genetic component was at work here, the researchers turned to the gene sequence that the bacterium uses when it obtain electrons from Fe(II). They knew that the proteins involved in the electron transfer from Fe(II) to the electron transport system are coded by the pioABC operon (1). The pioABC system, it turns out, also affects the electron uptake that was observed from the electrode. By inoculating strains of R. palustris to render them pioABC deficient (ΔpioABC) and then comparing these strains with the wild-type strains, they found that the mutant accepted 30% less current from the electrode than the wild-type strain in the light treatments. In addition, expression of certain pioABC proteins was upregulated in the wild-type strain during electron uptake. Protein expression of the pioABC was measured by its corresponding mRNA abundance. The researchers found that the pioA protein was upregulated the most in biofilms of R. palustris in illuminated conditions. pioA is a periplasmic decaheme cytochrome that is one of the key proteins responsible for ATP generation during electron transport (8), and so it is intuitive that this protein was regulated the most in the presence of light (Figure 5). This level of expression was comparable to the expression of pioC observed under photoferrotrophic growth on Fe(II) in a conventional culture apparatus. PioC is a periplasmic high potential iron-sulfur cluster protein. pioC expression was highest in the photoferrotrophic growth because the presence of iron stiumulated pioC to perform oxidation and reduction reactions. Expression of pioA was relatively low under these conditions, further suggesting that pioA is most active with the presence of light. Planktonic cells of R. palustris, which refer to cells that did not form biofilms on the electrode, exhibited lower levels of pio protein expression in illuminated conditions. As expected, the inoculum strain also exhibited very little expression. Together, this data suggests that the pioABC operon is one of the key molecular mechanisms responsible for extracellular electron transfer within R. palustris. It also indicates that the expression of certain proteins are favored when the bacteria has formed biofilms on the electrode. Biofilms and their relationship to extracellular transfer is addressed later on this page.
Expression of ruBisCO:
The researches utilized transcriptome analysis to sequence RNA strains of R. palustris to examine the role of the enzyme ruBisCO in electron uptake. RuBisCO is essential to all photosynthetic organisms because it is responsible for the conversion of carbon dioxide to energy-rich molecules like glucose (9). It does this by catalyzing the carboxylation of ribulose-1,5-bisphosphate, yielding two molecules of 3-phosphoglycerate which can then be reduced by NADPH in the Calvin cycle (1). R. palustris is able to express two different forms of ruBisCO, noted as Form I and II. Both forms perform similar functions and are responsible for the fixation of carbon dioxide, yet Girguis et al. found that form I was more highly expressed in the wild-type strain in the light treatments than form II (1). Also, expression of ruBisCO form I was significantly lower in the dark treatments. It has been known that in the presence of light, microbes absorb light and as a result produce ATP and NADPH. These energy carriers have been shown to positively regulate ruBisCO expression (9). In the dark, much less light can be absorbed by the microbe and so these energy-rich metabolites are much less abundant. If ATP and NADPH are low in abundance, ruBisCO expression will decrease.
R. palustris purposefully remains towards the surface of soil and water so that it can as close to sunlight as possible. This way, ruBisCO can be activated more regularly and electron uptake can occur. Yet the researches found that much of the ferrous minerals that it acquires electrons from lie deep within sediment because they are insoluble (1). In this way, R. palustris is unique among bacteria in its ability to access energy from both underground minerals and sunlight.
Nitrogen fixation and Hydrogen Production
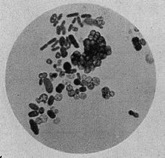
Another important aspect of R. palustris's metabolism is its highly efficient way of producing hydrogen. Hydrogen is a particularly efficient fuel source because it can be easily combined with oxygen to produce electricity, heat, and water. Many issues arise with using hydrogen as a primary fuel source to power automobiles and homes, one of them being that unless it is in liquid form, which requires energy to maintain, hydrogen gas takes up too much volume and can be hard to store (10). Nevertheless, hydrogen is produced as a byproduct of nitrogen fixation in many bacteria. Nitrogen-fixing bacteria use nitrogenases to convert nitrogen gas into ammonia, a usable form of nitrogen to plants (7). Nitrogenases also reduce protons to hydrogen gas (7). R. palustris is able to produce nearly three times as much hydrogen as most other nitrogen-fixing bacteria because it codes for an enzyme called vanadium nitrogenase (Figure 6). Vanadium nitrogenase is found in members of the Azotobacter but is also found in the species R. palustris and Anabaena variabilis (7). This differs from the more common nitrogen-fixing enzyme, molybdenum nitrogenase, in its ability to reduce more protons and thus generate more hydrogen gas. Along with cyanobacteria, R. palustris have been incorporated in microreactors to produce hydrogen that can be used for practical uses (5).
Electrical conductivity of biofilms
The formation of biofilms can play an important role in extracellular electron transfer. A biofilm is when a group of microorganisms stick to each other on a surface. Biofilms form for a number of reasons, but the primary reason microbes form biofilms is in response to a lack of nutrients (11). Biofilms also have unique properties with heat and electricity. Previously, it was known that biofilms could only withhold insulation (2). But a recent study done by Malvankar et al. (2012) on Geobacter sulfurreducens shows shows that both mixed-species and pure culture biofilms have the potential to conduct electricity. This is an important finding because it revealed that upon electron uptake, bacterium in a biofilm that were not in direct contact with an electrode contributed to current production equally as much as bacterium in direct contact. Thus, all bacterium in a biofilm have the potential to employ extracellular electron transfer, no matter how close they are to the direct source (2). This could potentially help explain why R. palustris was capable of extracting electrons from material deep within sediment.
Flavins and Quinones Enhance EET
In a study conducted by Akihiro Okamato et. al in 2012, researchers examined the role of secreted flavins in Shewanella oneidensis's metabolic processes. S. oneidensis is a gram-negative, anaerobically respiring bacterium that is often found in the deep-sea as well as soil and sedentary habitats (12). It is a member of the proteobacteria family and its genome is compacted within a single chromosome (12). S. oneidensis is another bacterium capable of extracellular electron transfer and it can use Fe(III) and Mn(IV)-oxide as its solid electron acceptors. The researchers were interested in how secreted flavins influence the metal reduction pathway, which is responsible for metal-oxide reduction reactions and the production of anodic current from the inner cellular membrane to the outer cellular membrane (3). Previous studies have showed that certain flavins, such as riboflavin and flavin mononucleotide, facilitate the metal reduction pathway by interacting with the OM protein MtrC (3). MtrC is a a 71-kDa decaheme c-Cytochrome that is responsible for catalyzing reactions within the metal reduction pathway and it is found on the outer membrane. Flavins are capable of increasing MtrC enzymatic activity by accessing heme centers on the exterior or buried within the protein scaffolds where they can serve as primary electron acceptors for the reduced hemes (3). Okamato et. al sought out to to further understand the mechanism by which flavins enhance the metal reduction pathway.
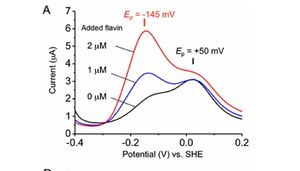
Similar to the study done by Girguis et. al, the researchers fabricated a monolayer biofilm of S. oneidensis on flat indium tin-oxide electrodes and used voltammetric techniques to monitor the redox properties of this species. Monolayer biofilms were exposed to increasing concentrations of flavin, and as expected, increased flavin concentration resulted in higher redox potentials within S. oneidensis cells (Figure 7). The researchers then studied flavin's interaction with the MtrC protein. It is important to note that when flavin (FMN) is fully oxidized, it can be reduced in either a one-electron reaction to form a derivative called semiquinone (sq), or a two-electron reaction to form hydroquinone (hq) (3). Semiquinone presence was measured in mutant strains of S. oneidensis lacking the ability to produce MtrC proteins (ΔmtrC) and OmcA proteins (ΔomcA). The ΔmtrC strain showed low levels of semiquinone formation, while the lack of OmcA proteins provided little effect on the formation of semiquinone. Taken together, these results suggest that the semiquinone formed in this reduction reaction is stabilized by the specific interaction with the MtrC protein (3).
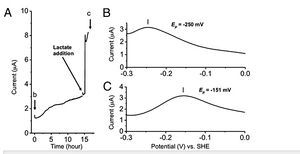
Semiquinone formation was also found to be highly coupled to S. oneidensis's metabolic activity. When grown with lactate as their main food source, cells are able to oxidize lactate and transfer the electrons to extracellular acceptors via the metal reduction pathway (3). Ultimately, the oxidation state of hemes in the protein reflect the balance between the electron input from respiration and the output due to extracellular electron transfer. Metabolically active cells require that most of the hemes in the MtrC protein are in a reduced state. In the absence of lactate, microbial current remained low. But when lactate was added to the culture, current increased (Figure 8) and the number of electrons of flavin reduction changed from 2 to 1. These findings allowed the researchers two make two very important conclusions. Microbial metabolism, which facilitates a continuous flow of electrons, is essential for this species of bacteria to use semiquinone as an enhancer of extracellular electron transfer. The other conclusion is that the activation of flavin is coupled to the nature of the oxidation state of the hemes in MtrC (3).
Various extracellular electron acceptors
From these two studies that addressed extracellular electron transfer, it is evident that the mechanisms behind this energy acquiring process is unique to the microbe. R. palustris was capable of extracellular electron transfer with ferrous iron has it's electron acceptor, but could also acquire electrons without it. S. oneidensis is capable of using Fe(III) and Mn(IV)-oxide as its solid electron acceptors. The range of solid-phase electron acceptors that are possible for extracellular electron transfer is quite extensive but has yet to be accurately determined. Many sulfate-reducing bacteria that use lactate as the electron donor can use hannebachite (CaSO3), gypsum (CaSO4), anglesite (PbSO4), all low-solubility crystallines (4). More research needs to be done on why certain solid-phase minerals serve as electron acceptors and why some cannot.
Conclusion
An advance in our understanding of R. palustris and S. oneidensis and how they undergo extracellular electron transfer has profound implications. Microbes that can perform electron extracellular transfer confer an ecological advantage – although soluble electron donors and acceptors are ubiquitous, the ability to acquire electrons from solid-phase materials can be beneficial in environments that are nutrient poor and uninhabitable to more basic microbes. Girguis’s study gave light to the possibility of using R. palustris in batteries or other practical appliances, although they were unsure that the bacterium would be a very efficient fuel source. If genes responsible for extracellular electron transfer were spliced into animal genomes or human genomes, could we acquire energy from our environment? This is a profound question that researchers are beginning to grapple with and explore.
References
1) Bose, A., Gardel, E.J., Girguis, P.R., Parra, E.A., Vidoudez, C., Electron uptake by iron-oxidizing phototrophic bacteria, Nature Communications, 2014, DOI: 10.1038/ncomms4391
2) Franks, A., Lau, J., Lovley, D., Malvankar, N., Nevin, K., Tuominen, M., Electrical Conductivity in a Mixed-Species Biofilm, Applied and Envrionmental Microbiology, 2012, Vol. 78, no.16 5967-5971.
3) Hashimoto, K., Nakamura, R., Nealson, K., Okamoto, A., Rate enhancement of bacterial extracellular electron transport involves bound flavin semiquinones, PNAS, 2012, DOI: 10.1073/pnas.1220823110.
4) Karnachuk OV1, Kurochkina SY, Tuovinen OH, Growth of sulfate-reducing bacteria with solid-phase electron acceptors, Pub Med, 2002.
5) Rhodopseudomonas palustris, http://en.wikipedia.org/wiki/Rhodopseudomonas_palustris, 2014
6) Rhodopseudomonas palustris and its role in bioremediation of soil pollutants, http://microbewiki.kenyon.edu/index.php/Rhodopseudomonas_palustris, 2014
7) Vanadium nitrogenase, http://en.wikipedia.org/wiki/Vanadium_nitrogenase, 2014
8) Cytochrome, http://en.wikipedia.org/wiki/Cytochrome, 2014
9) RuBisCO, http://en.wikipedia.org/wiki/RuBisCO, 2014
10) Hydrogen fuel, http://en.wikipedia.org/wiki/Hydrogen_fuel, 2014
11) Biofilm, http://en.wikipedia.org/wiki/Biofilm, 2014
12) Shewanella oneidensis, http://microbewiki.kenyon.edu/index.php/Shewanella_oneidensis, 2014