Pseudomonas syringae: Bioprecipitation Mechanisms and Implications: Difference between revisions
(55 intermediate revisions by 2 users not shown) | |||
Line 1: | Line 1: | ||
<!-- Do not edit this line-->{{Curated}} | <!-- Do not edit this line-->{{Curated}} | ||
==Overview== | ==Overview== | ||
<br>By: Brandon Byrd <br> | |||
<i>Pseudomonas syringae</i> have a significant impact on weather systems and ecosystems worldwide. This species of bacteria has previously been studied in depth as a plant pathogen, and it has recently been studied as a major contributor to bioprecipitation. <i>P. syringae</i> are rod shaped, gram-negative bacteria with polar flagella. They are also pathogens to a wide variety of plant species at cold temperatures [[#References|[10]]]. This bacterium is found in agricultural locations as well as non-agricultural locations such as clouds [[#References|[10]]]. The fact that <i>P. syringae</i> has the capability to grow in a wide range of environments and ecosystems gives this species of bacteria the potential to drastically impact biogeographical systems. <i>P. syringae</i> also has an extraordinary ice nucleation activity which allows this species to catalyze freezing water at warm temperatures which sparked interest in its role in the water cycle [[#References|[8]]]. This is a major key for <i>P. syringae’s</i> effect on biogeographical systems. It is also important to further analyze their ice nucleation ability to understand the mechanisms by which they influence major weather systems such as the water cycle. Furthermore, how <i>P. syringae</i> can impact humans and biodiversity based on their influence on ecosystems and the environment. | |||
< | ==History== | ||
[[Image:SEM_P.syringae.JPG|thumb|800px|right|Fig 1. Scanning Electron Microscopy of <i>Pseudomonas syringae</i> on an ovary of a tomato plant. <i>P.syringae</i> are rod shaped and gram-negative bacteria that possess polar flagella. http://www.apsnet.org/publications/phytopathology/backissues/Documents/1983Articles/Phyto73n01_39.PDF [[#References|[5]]]] | |||
<i> | The classification of the <i>P. syringae</i> species is important this bacterium's history. <i>P. syringae</i> are bacteria in the Proteobacteria phylum and the Gamma Proteobacteria class. This species belongs to the Pseudomonadales order and more specifically the Pseudomonadaceae family. Ultimately, <i>P. syringae</i> is classified in the Pseudomonas genus (Figure 1). Pseudomonads are gram-negative aerobic proteobacteria. This genus can be categorized as pathogens with a wide variety of niche potential due to much variation in metabolism throughout the genus. [[#References|[9]]]. The Pseudomonas genus is historically know to be important to aquatic ecosystems. | ||
The history of the Pseudomonas genus dates back millions of years ago to when they were found in aquatic habitats before plants and land agriculture [[#References|[9]]]. Pseudomonas is still one of the most common bacteria present in many aquatic environments today such as oceans and wetlands. Also, a study done by Pesciaroli[[#References|[14]]] and colleagues have shown a new strain of <i>P. syringae</i> to be present in intertidal aquatic environments [[#References|[9]]]. This new strain was intriguing since it demonstrated that these bacteria are capable of adapting to a variety of environments. Although this new strain of <i>P. syringae</i> was fascinating, this was not the first discovery of this species of bacteria. The original discovery of <i>P. syringae</i> revealed a special characteristic that this species possess. | |||
[[ | |||
The discovery of the <i>P. syringae</i> species was important in revealing these bacteria ice nucleation properties. <i>P. syringae</i> was first discovered by Paul Hoppe in 1961 while studying corn crops for the U.S. Department of Agriculture [[#References|[13]]]. His study demonstrated a bacteria that caused crop freezing problems at temperatures around negative two to four degrees Celsius. His research led to further research in later years which revealed <i>P. syringae</i> and its ice nucleation characteristics which were causing the crop freezing problems. The crop freezing problem that occurred is that between negative eight to four degrees plant stems and roots would freeze due to the presence of <i>P. syringae</i>. Ice nucleation research of <i>P. syringae</i> has led to promising discoveries that dig further into the mechanisms of <i>P. syringae's</i> ice nucleation abilities. | |||
There was a discovery of ice nucleation proteins that protrude from the outer membrane of <i>P. syringae</i> which facilitates their ice nucleation ability [[#References|[9]]]. These proteins are believed to have arisen without the use of horizontal gene transfer millions of years ago because of evidence of a three secretion system [[#References|[11]]]. Furthermore, these proteins are important for the mechanisms by which <i>P. syringae</i> conducts its ice nucleation. The gene for the outer membrane ice nucleation protein is most likely in the core genome of proteobacteria such as <i>Pseudomonas syringae</i>. The most likely common ancestor of this gene came from more general orders including Pseudomonadales and also Enterobacteriales [[#References|[9]]]. The study of these ice nucleation proteins have given rise to the present research regarding <i>P. syringae</i> in its direct significance to the water cycle and indirect significance to other biogeographical systems. | |||
. These proteins are believed to have arisen without the use of horizontal gene transfer millions of years ago because of evidence of a three secretion system | |||
. The gene for the outer membrane ice nucleation protein is most likely in the core genome of proteobacteria such as <i>Pseudomonas syringae</i>. The most likely common ancestor of this gene came from more general orders including Pseudomonadales and also Enterobacteriales | |||
. The study of these ice nucleation proteins have given rise to the present research regarding <i>P.syringae</i> in its direct significance to the water cycle and indirect significance to other biogeographical systems. | |||
==Bioprecipitation and <i>P.syringae’s</i> Role in Bioprecipitation== | ==Bioprecipitation and <i>P.syringae’s</i> Role in Bioprecipitation== | ||
[[Image:Project Pic.jpg|thumb|800px|left|Fig.2 The bioprecipitation cycle diagram with two key factors that highlight the system. First, micro-organisms such as <i>P.syringae</i> that conduct the ice nucleation process. Second, the water vapor from plants, oceans, and aquatic environments that these micro-organism use in the atmosphere. http://onlinelibrary.wiley.com/doi/10.1111/gcb.12447/epdf.]] | [[Image:Project Pic.jpg|thumb|800px|left|Fig.2 The bioprecipitation cycle diagram with two key factors that highlight the system. First, micro-organisms such as <i>P.syringae</i> that conduct the ice nucleation process. Second, the water vapor from plants, oceans, and aquatic environments that these micro-organism use in the atmosphere. http://onlinelibrary.wiley.com/doi/10.1111/gcb.12447/epdf [[#References|[12]]].]] | ||
Bioprecipitation is a term used to describe the concept in which precipitation is caused by bacteria. The bioprecipitation process can be compartmentalized and explained in a few steps within the water cycle (Figure 2). The first step is for ice nucleators such as <i>P. syringae</i> to become airborne in atmosphere ready to act on water vapor and ice nuclei at very low temperatures. The second step is for ice nucleation to occur using proteins that <i>P. syringae</i> possesses to facilitate this process. At this step cloud formation occurs and condensation of water vapor and ice nuclei takes place. The third step is traditional for the water cycle: the cloud becomes too heavy and gravity pulls the snow, rain, or any other form of precipitation from the cloud down to the Earth’s surface. This cycle continues which could potentially have a major impact on cloud formation and climate patterns. This is important because organisms such as bacteria that can drive our precipitation patterns, due to cloud formation, of any given area can be vital in predicting weather patterns. Also it deepen our understanding of the water cycle and how the water cycle effects ecosystems based on the makeup of the microbial community. This bacterium effects the environment and the weather of a given area due to its importance in the bioprecipitation. | |||
<i>P. syringae</i> plays an important role in bioprecipitation and its effects on the ecosystems and weather systems. Bioprecipitation has a strong correlation with <i>P. syringae</i>. <i>P. syringae</i> is thought to have two significant roles in cloud formation that effect bioprecipitation [[#References|[9]]]. One role is that due to its warm temperature ice nucleation activity, temperatures range from subzero to eight degrees Celsius. <i>P. syringae</i> is an organism that can cause water vapor droplets to freeze at temperatures and conditions that normal abundant mineral ice nucleator particles are unsuccessful at achieving. The second role that this bacteria causes water vapor to freeze to a large enough size for gravity to act on the droplets in clouds which causes precipitation such as snow or rain. This is important because much of rainfall that comes from mid-high altitudes are as result of this water droplet freezing process [[#References|[9]]]. This process either occurs by nucleation minerals present in clouds or microbial life such as <i>P. synrigae</i> that are also present in clouds. As described by in recent studies, <i>P. syringae</i> bioprecipitiation is constructed within the water cycle [#References|[2]]]. It was first discovered by a man named David Sands in the 1970s. Sands found the same pseudomonas bacteria that caused frost damage was also found in the rainfall of that area [[#References|[15]]]. Although it is clear that these bacteria contribute to bioprecipitation, the impact <i>P. syringae</i> and bioprecipitation has on the environment and the climate on a global scale is still debated. | |||
<i>P. syringae’s</i> global impact on ecosystems and weather systems are still unclear. It has been found that <i>P. syringae</i> only accounts for only a small fraction of the total microbial life deposited by rainfall [[#References|[2]]]. This makes it difficult to make the claim that bioprecipitation caused by <i>P. syringae</i> influences ecosystems on a global scale. However, research has suggested that <i>P. syringae</i> and other similar bacteria are unable to be cultured in high amounts via rainfall samples [[#References|[2]]]. This study also mentions that these ice nucleating bacteria still display high ice nucleation activity [[#References|[2]]]. These two claims create uncertainty regarding <i>P. syringae’s</i> significance on a global scale. In the future, better collection and culture techniques may be available in order to get a more accurate representation of these bacteria’s population levels in rain samples. Furthermore, this leaves more opportunity to explore how the rate of ice nucleation via <i>P. syringae</i> effects bioprecipitation despite their low atmospheric population discovered through precipitation samples. Also, the potential potency of <i>P. syringae</i> increases because they are able to grow in a wide range of environments [[#References|[12]]]. This bacteria’s life cycle can be conducted in air-borne environments, as well as soil and non-air-borne environments. Other microbial species’ life cycle are heavily dependent on air-borne distribution and deposition through rainfall. These species have recently been shown to be high in ice nucleation activity as well [[#References|[12]]]. This is noteworthy because more microbes other than <i>P. syringae</i> may have a high impact on bioprecipitation. This could also potentially explain the low population numbers of <i>P. syringae</i> in precipitation samples. A deeper understanding of <i>P. syringae’s</i> protein structure and function regarding ice nucleation is important to expanding knowledge on bioprecipitation as a whole. | |||
<br> | <br> | ||
==Mechanisms for Ice Nucleation== | ==Mechanisms for Ice Nucleation== | ||
[[Image:InaZ_Figure.JPG|thumb|500px|right|Fig.3 Ice nucleation activity of <i>P. | [[Image:InaZ_Figure.JPG|thumb|500px|right|Fig.3 Ice nucleation activity of <i>P. syringae</i> and <i>E. coli</i> cells in the inner and outer membrane at high ice nucleation temperatures. Different shapes indicate different treatments: wild type <i>P. syringae</i> (X), <i>E. coli</i> with InaZ proteins (+), <i>E. coli</i> with inner membrane InaZ proteins (square), <i>E. coli</i> with outer membrane InaZ proteins (triangle) , <i>E. coli</i> with abnormally dense concentrations of InaZ protein (diamond), and cell extract from dense InaZ protein <i>E. coli </i> treatment (O). http://www.pnas.org/content/83/19/7256.full.pdf[[#References|[17]]].]] | ||
<i>P. syringae</i> ice nucleation is due to the proteins coded by a specific gene. These bacteria’s ice nucleation protein is known as lnaZ. Studies have proven that the lnaZ protein is coded by the p153 gene in <i>P. syringae</i> [[#References|[17]]]. <i>P. syringae</i> with functioning InaZ proteins have a genotype of InaZ+ whereas <i>P. syringae</i> without functioning InaZ have a genotype of InaZ-. Wolber and colleagues [[#References|[17]]] proved that p153 is indeed the gene that codes for the InaZ protein by identifying this gene’s N-terminal Edman sequence. The sequence observed was composed of the following: Methionine-Asparagine-Leucine-Aspartic Acid-Lysine-Alanine-Leucine-Valine-Leucine. This sequence was in perfect agreement with the sequence predicted from inaZ. Therefore, they determined that p153 was the gene that codes for the InaZ protein. Determining the gene that codes for a specific protein is important but the structure of a protein is essential as well. | |||
The structure of InaZ has been a constant area of research. Model simulations have proposed the structure of ice nucleation proteins such as InaZ to have one side designated to organizing water molecules into an ice structure, and the other side of the protein designated to binding to the cell membrane of the bacteria [[#References|[7]]]. More recent studies have shown that there are significant greater amounts of beta-sheets than alpha-helices in quaternary protein structure [[#References|[6]]]. The significance of this has yet to be determined. However, these results produce an intriguing area of research since increasing our knowledgebase of the InaZ protein structure influencing its function will aid in understanding the mechanisms of InaZ proteins. | |||
The main function of the InaZ protein is to participate in ice crystallization of pure water. Researchers have determined that there is no evidence that the ice nucleation protein serves any function other than ice nucleation; therefore, the positive natural selection of the InaZ protein is based on the ability of an organism to ice nucleate [[#References|[12]]]. There are two types of crystallization: homogeneous and heterogeneous. Homogeneous crystallization occurs when the right conditions-negative eight to four degree temperature range, water vapor, atmospheric pressure- are reached for the formation of ice due to a group of water molecules to orientate their particles into a certain order [[#References|[17]]]. This type of crystallization is also known as ice nuclei crystallization. Heterogeneous crystallization is when solid, non-water molecules, are used for ice formation sites [[#References|[17]]]. <i>P. syringae</i> InaZ proteins conduct homogenous or ice nuclei crystallization. Wolber and colleagues also found a common repeating Alanine-Glycine-Tyrosine-Glycine-Serine-Threonine-Leucine pattern within a 1200 amino acid sequence in the InaZ protein complex [[#References|[17]]]. This sequence is considered to be responsible for water molecule organization due to alterations in spatial location and alignment prompting ice formation [[#References|[3]]]. The efficiency of the InaZ protein is also an important area of research. | |||
The efficiency of the ice crystallization depends on the environmental conditions, protein location, and cell concentration. The most favorable environment for optimal <i>P. syringae</i> is a high water vapor environment, although there has been a water vapor threshold or capacity where efficiency becomes independent of the concentration [[#References|[17]]]. However, InaZ have been shown to be more efficient than other ice nucleators such as other fungi species and ash, dust, soot, and other ice nucleation minerals [[#References|[12]]]. One proposed reason for this is that <i>P. syringae</i> InaZ proteins have more amounts of ice active sites per surface area of their cells in fungi InaZ proteins. The mechanisms of InaZ gene to protein pathways and InaZ protein function are still being investigated in the present day. The location of these proteins are typically found in both the inner and outer membrane of these bacteria. In this study, the InaZ protein was researched through the use of <i>Escherichia coli</i> bacteria. The <i>E. coli</i> bacteria in this study were either InaZ- -control group-, or displayed an over expression of the p153 gene causing them to be InaZ+. One focus of this study was to determine how the location and density of these proteins effect ice nuclei activity (Figure 3). This study revealed ice nuclei activity was high in both inner and outer membrane <i>E. coli</i> groups. Conversely, the <i>E. coli</i> group with the abnormally high InaZ protein density indeed had the highest ice nuclei activity. These results show that the location and density of InaZ proteins are determinants of the efficiency of these proteins. | |||
==Ecological Implications of <br><i>P.syringae</i> Bioprecipitation== | ==Ecological Implications of <br><i>P.syringae</i> Bioprecipitation== | ||
<br> | <br> | ||
[[Image:Nitrogen Triangle.jpg|thumb|500px|left|Fig 4. The “Nitrogen Triangle” breaking down the three major parts of the nitrogen cycle. Also the important molecules at each step are included. http:// | [[Image:Nitrogen Triangle.jpg|thumb|500px|left|Fig 4. The “Nitrogen Triangle” breaking down the three major parts of the nitrogen cycle. Also the important molecules at each step are included. http://books.wwnorton.com/books/webad.aspx?id=4294977366 (Chapter 22.4 + Figure 22.11A) [[#References|[16]]].]] | ||
<i>P.syringae</i> | <i>P. syringae's</i> ability to survive in a wide variety of environments can impact ecosystems on a biotic and abiotic level. These bacteria are fairly abundant in nature. <i>P. syringae</i> has been found in a wide range of environments that have favorable substrates to the water cycle. These include plant canopies, clouds, snow and rainfall, leaf litter, lakes, oceans, rivers, and biofilms associated with wild and cultured plants [[#References|[9]]]. This is significant since these locations are high in water and water vapor content. Therefore, <i>P. syringae</i> is very important for recycling water in many different ecosystems due to its ice nucleation abilities. It has the potential to change the amount of precipitation in a given ecosystem which can possibly limit plant diversity based on water level tolerances of a given plant species. Also, if there are less <i>P. syringae</i> in the atmosphere then the amount of water vapor increases since the vapor is not being condensed and frozen into potential precipitation from clouds. Both of these factors can control the diversity of ecosystems that depend on <i>P. syringae</i> to drive a significant part of their regional water cycle. This diversity dynamic not only effects plant species but also other animal species at higher trophic levels that depend on these plants for energy. Less plant diversity could lead to less available energy in a given system if other plant species are not able to fill previous plants’ ecological niches. This is logical reasoning because the science community knows that plants are the primary producers in a trophic web ecosystem. Therefore, reduction or addition of more plants into an ecosystem can alter the amount of available energy in a system, which causes a cascade of effects. | ||
Ecologcial effects caused by <i>P.syringae’s</i> impact on the water cycle may bleed into other important natural cycles and biodiversity. For example, if there is more precipitation in a heavily agricultural based area such as a farm, this can lead to more nitrogen runoff into water systems such as nearby rivers and lakes. Therefore, the population numbers of an ice nucleating organism like <i>P.syringae</i> can have a profound effect on the nitrogen cycle. The nitrogen cycle can be broken into three sections of the organization model known as the nitrogen triangle (Figure 4). High populations can accelerate the nitrification phase of the cycle in the nitrogen triangle and decelerate denitrification of oxidized compounds returning to atmospheric form. This happens because the higher populations of <i>P.syringae</i> in the atmosphere could lead to precipitation increases due to their ice nucleation ability via the InaZ protein | Ecologcial effects caused by <i>P. syringae’s</i> impact on the water cycle may bleed into other important natural cycles and biodiversity. For example, if there is more precipitation in a heavily agricultural based area such as a farm, this can lead to more nitrogen runoff into water systems such as nearby rivers and lakes. Therefore, the population numbers of an ice nucleating organism like <i>P. syringae</i> can have a profound effect on the nitrogen cycle. The nitrogen cycle can be broken into three sections of the organization model known as the nitrogen triangle (Figure 4). High populations can accelerate the nitrification phase of the cycle in the nitrogen triangle and decelerate denitrification of oxidized compounds returning to atmospheric form. This happens because the higher populations of <i>P. syringae</i> in the atmosphere could lead to precipitation increases due to their ice nucleation ability via the InaZ protein [[#References|[9]]]. Consequently, increasing in the amount of nitrogen runoff into local water systems from farm fertilizers and thereby causing reactive nitrogen levels in these water systems to increase. This not only effects the nitrogen cycle, but it also affects the organisms that live in these lakes and streams. | ||
Nitrogen level | Nitrogen level changes to marine ecosystems can be linked by to <i>P. syringae</i> population levels. In the aquatic ecosystem, reactive deposition into primarily fresh water ecosystems can increase the acidity of the water [[#References|[4]]]. Constant acidification of the water systems can cause the ecosystem to favor acid tolerant organisms such as phytoplankton. This changes the food chain dynamic of the ecosystem because many fish and invertebrates in aquatic ecosystems are sensitive to acidification. Thus, resulting in a decrease in their population levels [[#References|[4]]]. Acidification also has also been shown to effect organisms at higher trophic levels such as birds, zooplankton, amphibians, and benthic invertebrates directly and indirectly [[#References|[4]]]. Eutrophication can be a result of higher nitrogen levels into marine ecosystems via fertilizer runoff. Local water systems such as lakes are typically low in nitrogen nutrients. However, the introduction of nitrogen to the water system can cause the nutrients to increase. Organisms like phytoplankton that are more effective in assimilating these nutrients are better suited for these conditions over species limited by other factors [[#References|[4]]]. For example, diatoms’ limiting factor is silica and benthic plants are limited by light availability. The higher nutrients in the water can cause algal blooms by cyanobacteria which can cover the surface water of lakes. This can then lead to hypoxia at the surface when deadly toxins are released creating dead zones [[#References|[4]]]. This again can change the effect on higher trophic organisms such as fish and invertebrates. Furthermore, in ecosystems with minimal water turnover- lakes for example- the sedimentation and breakdown of the organic biomass from algal blooms in the water causes oxygen depletion in the water [[#References|[4]]]. This further changes biodiversity in the ecosystem to favor more oxygen tolerant species which are low in population number. Constant changes to the biodiversity can alter nutrient cycling in the aquatic ecosystem which changes the ecosystem as a whole [[#References|[4]]]. These ecological alterations can be looped back to the presence of <i>P. syringae</i> levels in a given area. Since these changes stem from agricultural runoff which is controlled by precipitation level, <i>P. syringae</i> is important because it is one of the regulators of precipitation and cloud formation. | ||
Changes in land use could also influence the bioprecipitation cycle causing climate fluctuations and changes to ice nucleating organisms like <i>P.syringaes’</i> future role in this cycle. Modifications to the vegetation density and type have been shown to change the source of ice nucleating organisms released into the atmosphere, therefore impacting cloud formation, cloud patterns, and precipitation patterns | Changes in land use could also influence the bioprecipitation cycle causing climate fluctuations and changes to ice nucleating organisms like <i>P. syringaes’</i> future role in this cycle. Modifications to the vegetation density and type have been shown to change the source of ice nucleating organisms released into the atmosphere, therefore impacting cloud formation, cloud patterns, and precipitation patterns [[#References|[12]]]. This results in fluctuations in the amount of radiation entering the upper atmosphere and hitting the Earth’s surface. This causes possible effects on the regional climate. A change in regional climate can affect agricultural methods for farmers since crops grow more productively at particular temperatures. Thus, impacting what kinds of crops can be grown in different regions and ultimately effecting the yield farms collect per growing season. Change in regional climate can also change the diversity of life within the regions ecosystem for similar reasoning. Organisms are known to be affected by climate change. This is because organisms such as macroinvertbrates have thermal neutral zones [[#References|[1]]]. Thermal neutral zones are the tolerance ranges of a specific species of organisms. Therefore, fluctuations and changes in climate can alter the biodiversity in a given region since climate changes may force some species to operate outside their climate tolerance. When this occurs these species may die off, therefore reducing the biodiversity in the region. However, climate change may increase the populations of endemic species or provide ecological opportunity for new species. This is because the change organisms that optimally operate at the new temperatures, due to the climate change, will thrive and reproduce. The net effect of the climate change will then be a reduction, if new species do not replace endemic species, or a change in biodiversity, if new species do replace endemic species. These phenomena demonstrate <i>P. syringaes’</i> importance to agricultural methods and biodiversity on a regional scale. | ||
==Conclusion== | ==Conclusion== | ||
Line 56: | Line 61: | ||
<references /> | <references /> | ||
<br><br>Authored for BIOL 238 Microbiology, taught by [mailto:slonczewski@kenyon.edu Joan Slonczewski], 2016, [http://www.kenyon.edu/index.xml Kenyon College]. | <br><br>Authored for BIOL 238 Microbiology, taught by [mailto:slonczewski@kenyon.edu Joan Slonczewski], 2016, [http://www.kenyon.edu/index.xml Kenyon College]. | ||
<br> | |||
1.Burgmer, T. et al. 2007. Effects of climate-driven temperature changes on the diversity of freshwater macroinvertebrates. Oecologia Vol. 151: 93-103. | 1.Burgmer, T. et al. 2007. Effects of climate-driven temperature changes on the diversity of freshwater macroinvertebrates. Oecologia Vol. 151: 93-103. | ||
http://download.springer.com/static/pdf/405/art%253A10.1007%252Fs00442-006-0542-9.pdf?originUrl=http%3A%2F%2Flink.springer.com%2Farticle%2F10.1007%2Fs00442-006-0542-9&token2=exp=1461612065~acl=%2Fstatic%2Fpdf%2F405%2Fart%25253A10.1007%25252Fs00442-006-0542-9.pdf%3ForiginUrl%3Dhttp%253A%252F%252Flink.springer.com%252Farticle%252F10.1007%252Fs00442-006-0542-9*~hmac=1d0eca244036a3758432a3ddd64c9a7bd8d9da38d61bc77ae9a6a025422223a7 | http://download.springer.com/static/pdf/405/art%253A10.1007%252Fs00442-006-0542-9.pdf?originUrl=http%3A%2F%2Flink.springer.com%2Farticle%2F10.1007%2Fs00442-006-0542-9&token2=exp=1461612065~acl=%2Fstatic%2Fpdf%2F405%2Fart%25253A10.1007%25252Fs00442-006-0542-9.pdf%3ForiginUrl%3Dhttp%253A%252F%252Flink.springer.com%252Farticle%252F10.1007%252Fs00442-006-0542-9*~hmac=1d0eca244036a3758432a3ddd64c9a7bd8d9da38d61bc77ae9a6a025422223a7 | ||
2.Christner, Brent C. "Cloudy With a Chance of Microbes." Microbe 7.2 (2012): 70-75. Christner Research Group. Louisiana State University. Web. 28 Oct. 2012. | 2.Christner, Brent C. "Cloudy With a Chance of Microbes." Microbe 7.2 (2012): 70-75. Christner Research Group. Louisiana State University. Web. 28 Oct. 2012. | ||
http://brent.xner.net/pdf/Christner2012_CloudyMicrobes.pdf | http://brent.xner.net/pdf/Christner2012_CloudyMicrobes.pdf | ||
Line 80: | Line 87: | ||
9.Morris, C. E. et al. 2013. The life history of Pseudomonas syringae: linking agriculture to earth system processes. Annu. Rev. Phytopathol. Vol. 51: 85–104 | 9.Morris, C. E. et al. 2013. The life history of Pseudomonas syringae: linking agriculture to earth system processes. Annu. Rev. Phytopathol. Vol. 51: 85–104 | ||
http://www.annualreviews.org/doi/pdf/10.1146/annurev-phyto-082712-102402 | http://www.annualreviews.org/doi/pdf/10.1146/annurev-phyto-082712-102402 | ||
Line 99: | Line 105: | ||
http://wsbs-msu.ru/res/DictionaryAttachment/602/DOC_FILENAME/C%20Pesciaroli%20et%20al%202012%20Termperature%20preferences%20of%20bacteria%20isolated%20from%20seawater%20collected%20in%20Kandaklaksha%20Bay.pdf | http://wsbs-msu.ru/res/DictionaryAttachment/602/DOC_FILENAME/C%20Pesciaroli%20et%20al%202012%20Termperature%20preferences%20of%20bacteria%20isolated%20from%20seawater%20collected%20in%20Kandaklaksha%20Bay.pdf | ||
15.Prasanthm M, et al. 2015. Pseudomonas syringae: An overview and its future as a “rain making bacteria”. International Research Journal of Biological Sciences Vol. 4(2): 70-77. | 15.Prasanthm M, et al. 2015. Pseudomonas syringae: An overview and its future as a “rain making bacteria”. International Research Journal of Biological Sciences Vol. 4(2): 70-77. http://www.isca.in/IJBS/Archive/v4/i2/13.ISCA-IRJBS-2014-229.pdf | ||
16.Slonczewski, Joan L., and John Watkins. Foster. Microbiology: An Evolving Science. First ed. New York: W.W. Norton Et, 2009. Print. Chapter 22.4. Figure 22.11A. | |||
http://books.wwnorton.com/books/webad.aspx?id=4294977366 | |||
17.Wolber, P.K. et al. 1986. Identification and purification of a bacterial ice-nucleation protein. Proc. Natl. Acad. Sci. USA. Vol. 83, pp. 7256-7260. http://www.pnas.org/content/83/19/7256.full.pdf | |||
17. Wolber, P.K. et al. 1986. Identification and purification of a bacterial ice-nucleation protein. Proc. Natl. Acad. Sci. USA. Vol. 83, pp. 7256-7260. http://www.pnas.org/content/83/19/7256.full.pdf |
Latest revision as of 18:48, 9 May 2016
Overview
By: Brandon Byrd
Pseudomonas syringae have a significant impact on weather systems and ecosystems worldwide. This species of bacteria has previously been studied in depth as a plant pathogen, and it has recently been studied as a major contributor to bioprecipitation. P. syringae are rod shaped, gram-negative bacteria with polar flagella. They are also pathogens to a wide variety of plant species at cold temperatures [10]. This bacterium is found in agricultural locations as well as non-agricultural locations such as clouds [10]. The fact that P. syringae has the capability to grow in a wide range of environments and ecosystems gives this species of bacteria the potential to drastically impact biogeographical systems. P. syringae also has an extraordinary ice nucleation activity which allows this species to catalyze freezing water at warm temperatures which sparked interest in its role in the water cycle [8]. This is a major key for P. syringae’s effect on biogeographical systems. It is also important to further analyze their ice nucleation ability to understand the mechanisms by which they influence major weather systems such as the water cycle. Furthermore, how P. syringae can impact humans and biodiversity based on their influence on ecosystems and the environment.
History
The classification of the P. syringae species is important this bacterium's history. P. syringae are bacteria in the Proteobacteria phylum and the Gamma Proteobacteria class. This species belongs to the Pseudomonadales order and more specifically the Pseudomonadaceae family. Ultimately, P. syringae is classified in the Pseudomonas genus (Figure 1). Pseudomonads are gram-negative aerobic proteobacteria. This genus can be categorized as pathogens with a wide variety of niche potential due to much variation in metabolism throughout the genus. [9]. The Pseudomonas genus is historically know to be important to aquatic ecosystems.
The history of the Pseudomonas genus dates back millions of years ago to when they were found in aquatic habitats before plants and land agriculture [9]. Pseudomonas is still one of the most common bacteria present in many aquatic environments today such as oceans and wetlands. Also, a study done by Pesciaroli[14] and colleagues have shown a new strain of P. syringae to be present in intertidal aquatic environments [9]. This new strain was intriguing since it demonstrated that these bacteria are capable of adapting to a variety of environments. Although this new strain of P. syringae was fascinating, this was not the first discovery of this species of bacteria. The original discovery of P. syringae revealed a special characteristic that this species possess.
The discovery of the P. syringae species was important in revealing these bacteria ice nucleation properties. P. syringae was first discovered by Paul Hoppe in 1961 while studying corn crops for the U.S. Department of Agriculture [13]. His study demonstrated a bacteria that caused crop freezing problems at temperatures around negative two to four degrees Celsius. His research led to further research in later years which revealed P. syringae and its ice nucleation characteristics which were causing the crop freezing problems. The crop freezing problem that occurred is that between negative eight to four degrees plant stems and roots would freeze due to the presence of P. syringae. Ice nucleation research of P. syringae has led to promising discoveries that dig further into the mechanisms of P. syringae's ice nucleation abilities.
There was a discovery of ice nucleation proteins that protrude from the outer membrane of P. syringae which facilitates their ice nucleation ability [9]. These proteins are believed to have arisen without the use of horizontal gene transfer millions of years ago because of evidence of a three secretion system [11]. Furthermore, these proteins are important for the mechanisms by which P. syringae conducts its ice nucleation. The gene for the outer membrane ice nucleation protein is most likely in the core genome of proteobacteria such as Pseudomonas syringae. The most likely common ancestor of this gene came from more general orders including Pseudomonadales and also Enterobacteriales [9]. The study of these ice nucleation proteins have given rise to the present research regarding P. syringae in its direct significance to the water cycle and indirect significance to other biogeographical systems.
Bioprecipitation and P.syringae’s Role in Bioprecipitation
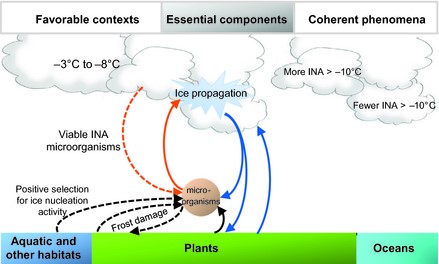
Bioprecipitation is a term used to describe the concept in which precipitation is caused by bacteria. The bioprecipitation process can be compartmentalized and explained in a few steps within the water cycle (Figure 2). The first step is for ice nucleators such as P. syringae to become airborne in atmosphere ready to act on water vapor and ice nuclei at very low temperatures. The second step is for ice nucleation to occur using proteins that P. syringae possesses to facilitate this process. At this step cloud formation occurs and condensation of water vapor and ice nuclei takes place. The third step is traditional for the water cycle: the cloud becomes too heavy and gravity pulls the snow, rain, or any other form of precipitation from the cloud down to the Earth’s surface. This cycle continues which could potentially have a major impact on cloud formation and climate patterns. This is important because organisms such as bacteria that can drive our precipitation patterns, due to cloud formation, of any given area can be vital in predicting weather patterns. Also it deepen our understanding of the water cycle and how the water cycle effects ecosystems based on the makeup of the microbial community. This bacterium effects the environment and the weather of a given area due to its importance in the bioprecipitation.
P. syringae plays an important role in bioprecipitation and its effects on the ecosystems and weather systems. Bioprecipitation has a strong correlation with P. syringae. P. syringae is thought to have two significant roles in cloud formation that effect bioprecipitation [9]. One role is that due to its warm temperature ice nucleation activity, temperatures range from subzero to eight degrees Celsius. P. syringae is an organism that can cause water vapor droplets to freeze at temperatures and conditions that normal abundant mineral ice nucleator particles are unsuccessful at achieving. The second role that this bacteria causes water vapor to freeze to a large enough size for gravity to act on the droplets in clouds which causes precipitation such as snow or rain. This is important because much of rainfall that comes from mid-high altitudes are as result of this water droplet freezing process [9]. This process either occurs by nucleation minerals present in clouds or microbial life such as P. synrigae that are also present in clouds. As described by in recent studies, P. syringae bioprecipitiation is constructed within the water cycle [#References|[2]]]. It was first discovered by a man named David Sands in the 1970s. Sands found the same pseudomonas bacteria that caused frost damage was also found in the rainfall of that area [15]. Although it is clear that these bacteria contribute to bioprecipitation, the impact P. syringae and bioprecipitation has on the environment and the climate on a global scale is still debated.
P. syringae’s global impact on ecosystems and weather systems are still unclear. It has been found that P. syringae only accounts for only a small fraction of the total microbial life deposited by rainfall [2]. This makes it difficult to make the claim that bioprecipitation caused by P. syringae influences ecosystems on a global scale. However, research has suggested that P. syringae and other similar bacteria are unable to be cultured in high amounts via rainfall samples [2]. This study also mentions that these ice nucleating bacteria still display high ice nucleation activity [2]. These two claims create uncertainty regarding P. syringae’s significance on a global scale. In the future, better collection and culture techniques may be available in order to get a more accurate representation of these bacteria’s population levels in rain samples. Furthermore, this leaves more opportunity to explore how the rate of ice nucleation via P. syringae effects bioprecipitation despite their low atmospheric population discovered through precipitation samples. Also, the potential potency of P. syringae increases because they are able to grow in a wide range of environments [12]. This bacteria’s life cycle can be conducted in air-borne environments, as well as soil and non-air-borne environments. Other microbial species’ life cycle are heavily dependent on air-borne distribution and deposition through rainfall. These species have recently been shown to be high in ice nucleation activity as well [12]. This is noteworthy because more microbes other than P. syringae may have a high impact on bioprecipitation. This could also potentially explain the low population numbers of P. syringae in precipitation samples. A deeper understanding of P. syringae’s protein structure and function regarding ice nucleation is important to expanding knowledge on bioprecipitation as a whole.
Mechanisms for Ice Nucleation
P. syringae ice nucleation is due to the proteins coded by a specific gene. These bacteria’s ice nucleation protein is known as lnaZ. Studies have proven that the lnaZ protein is coded by the p153 gene in P. syringae [17]. P. syringae with functioning InaZ proteins have a genotype of InaZ+ whereas P. syringae without functioning InaZ have a genotype of InaZ-. Wolber and colleagues [17] proved that p153 is indeed the gene that codes for the InaZ protein by identifying this gene’s N-terminal Edman sequence. The sequence observed was composed of the following: Methionine-Asparagine-Leucine-Aspartic Acid-Lysine-Alanine-Leucine-Valine-Leucine. This sequence was in perfect agreement with the sequence predicted from inaZ. Therefore, they determined that p153 was the gene that codes for the InaZ protein. Determining the gene that codes for a specific protein is important but the structure of a protein is essential as well.
The structure of InaZ has been a constant area of research. Model simulations have proposed the structure of ice nucleation proteins such as InaZ to have one side designated to organizing water molecules into an ice structure, and the other side of the protein designated to binding to the cell membrane of the bacteria [7]. More recent studies have shown that there are significant greater amounts of beta-sheets than alpha-helices in quaternary protein structure [6]. The significance of this has yet to be determined. However, these results produce an intriguing area of research since increasing our knowledgebase of the InaZ protein structure influencing its function will aid in understanding the mechanisms of InaZ proteins.
The main function of the InaZ protein is to participate in ice crystallization of pure water. Researchers have determined that there is no evidence that the ice nucleation protein serves any function other than ice nucleation; therefore, the positive natural selection of the InaZ protein is based on the ability of an organism to ice nucleate [12]. There are two types of crystallization: homogeneous and heterogeneous. Homogeneous crystallization occurs when the right conditions-negative eight to four degree temperature range, water vapor, atmospheric pressure- are reached for the formation of ice due to a group of water molecules to orientate their particles into a certain order [17]. This type of crystallization is also known as ice nuclei crystallization. Heterogeneous crystallization is when solid, non-water molecules, are used for ice formation sites [17]. P. syringae InaZ proteins conduct homogenous or ice nuclei crystallization. Wolber and colleagues also found a common repeating Alanine-Glycine-Tyrosine-Glycine-Serine-Threonine-Leucine pattern within a 1200 amino acid sequence in the InaZ protein complex [17]. This sequence is considered to be responsible for water molecule organization due to alterations in spatial location and alignment prompting ice formation [3]. The efficiency of the InaZ protein is also an important area of research.
The efficiency of the ice crystallization depends on the environmental conditions, protein location, and cell concentration. The most favorable environment for optimal P. syringae is a high water vapor environment, although there has been a water vapor threshold or capacity where efficiency becomes independent of the concentration [17]. However, InaZ have been shown to be more efficient than other ice nucleators such as other fungi species and ash, dust, soot, and other ice nucleation minerals [12]. One proposed reason for this is that P. syringae InaZ proteins have more amounts of ice active sites per surface area of their cells in fungi InaZ proteins. The mechanisms of InaZ gene to protein pathways and InaZ protein function are still being investigated in the present day. The location of these proteins are typically found in both the inner and outer membrane of these bacteria. In this study, the InaZ protein was researched through the use of Escherichia coli bacteria. The E. coli bacteria in this study were either InaZ- -control group-, or displayed an over expression of the p153 gene causing them to be InaZ+. One focus of this study was to determine how the location and density of these proteins effect ice nuclei activity (Figure 3). This study revealed ice nuclei activity was high in both inner and outer membrane E. coli groups. Conversely, the E. coli group with the abnormally high InaZ protein density indeed had the highest ice nuclei activity. These results show that the location and density of InaZ proteins are determinants of the efficiency of these proteins.
Ecological Implications of
P.syringae Bioprecipitation
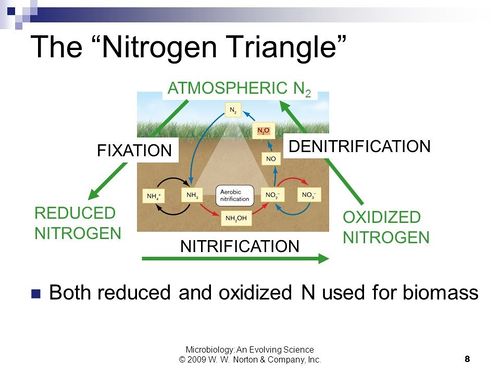
P. syringae's ability to survive in a wide variety of environments can impact ecosystems on a biotic and abiotic level. These bacteria are fairly abundant in nature. P. syringae has been found in a wide range of environments that have favorable substrates to the water cycle. These include plant canopies, clouds, snow and rainfall, leaf litter, lakes, oceans, rivers, and biofilms associated with wild and cultured plants [9]. This is significant since these locations are high in water and water vapor content. Therefore, P. syringae is very important for recycling water in many different ecosystems due to its ice nucleation abilities. It has the potential to change the amount of precipitation in a given ecosystem which can possibly limit plant diversity based on water level tolerances of a given plant species. Also, if there are less P. syringae in the atmosphere then the amount of water vapor increases since the vapor is not being condensed and frozen into potential precipitation from clouds. Both of these factors can control the diversity of ecosystems that depend on P. syringae to drive a significant part of their regional water cycle. This diversity dynamic not only effects plant species but also other animal species at higher trophic levels that depend on these plants for energy. Less plant diversity could lead to less available energy in a given system if other plant species are not able to fill previous plants’ ecological niches. This is logical reasoning because the science community knows that plants are the primary producers in a trophic web ecosystem. Therefore, reduction or addition of more plants into an ecosystem can alter the amount of available energy in a system, which causes a cascade of effects.
Ecologcial effects caused by P. syringae’s impact on the water cycle may bleed into other important natural cycles and biodiversity. For example, if there is more precipitation in a heavily agricultural based area such as a farm, this can lead to more nitrogen runoff into water systems such as nearby rivers and lakes. Therefore, the population numbers of an ice nucleating organism like P. syringae can have a profound effect on the nitrogen cycle. The nitrogen cycle can be broken into three sections of the organization model known as the nitrogen triangle (Figure 4). High populations can accelerate the nitrification phase of the cycle in the nitrogen triangle and decelerate denitrification of oxidized compounds returning to atmospheric form. This happens because the higher populations of P. syringae in the atmosphere could lead to precipitation increases due to their ice nucleation ability via the InaZ protein [9]. Consequently, increasing in the amount of nitrogen runoff into local water systems from farm fertilizers and thereby causing reactive nitrogen levels in these water systems to increase. This not only effects the nitrogen cycle, but it also affects the organisms that live in these lakes and streams.
Nitrogen level changes to marine ecosystems can be linked by to P. syringae population levels. In the aquatic ecosystem, reactive deposition into primarily fresh water ecosystems can increase the acidity of the water [4]. Constant acidification of the water systems can cause the ecosystem to favor acid tolerant organisms such as phytoplankton. This changes the food chain dynamic of the ecosystem because many fish and invertebrates in aquatic ecosystems are sensitive to acidification. Thus, resulting in a decrease in their population levels [4]. Acidification also has also been shown to effect organisms at higher trophic levels such as birds, zooplankton, amphibians, and benthic invertebrates directly and indirectly [4]. Eutrophication can be a result of higher nitrogen levels into marine ecosystems via fertilizer runoff. Local water systems such as lakes are typically low in nitrogen nutrients. However, the introduction of nitrogen to the water system can cause the nutrients to increase. Organisms like phytoplankton that are more effective in assimilating these nutrients are better suited for these conditions over species limited by other factors [4]. For example, diatoms’ limiting factor is silica and benthic plants are limited by light availability. The higher nutrients in the water can cause algal blooms by cyanobacteria which can cover the surface water of lakes. This can then lead to hypoxia at the surface when deadly toxins are released creating dead zones [4]. This again can change the effect on higher trophic organisms such as fish and invertebrates. Furthermore, in ecosystems with minimal water turnover- lakes for example- the sedimentation and breakdown of the organic biomass from algal blooms in the water causes oxygen depletion in the water [4]. This further changes biodiversity in the ecosystem to favor more oxygen tolerant species which are low in population number. Constant changes to the biodiversity can alter nutrient cycling in the aquatic ecosystem which changes the ecosystem as a whole [4]. These ecological alterations can be looped back to the presence of P. syringae levels in a given area. Since these changes stem from agricultural runoff which is controlled by precipitation level, P. syringae is important because it is one of the regulators of precipitation and cloud formation.
Changes in land use could also influence the bioprecipitation cycle causing climate fluctuations and changes to ice nucleating organisms like P. syringaes’ future role in this cycle. Modifications to the vegetation density and type have been shown to change the source of ice nucleating organisms released into the atmosphere, therefore impacting cloud formation, cloud patterns, and precipitation patterns [12]. This results in fluctuations in the amount of radiation entering the upper atmosphere and hitting the Earth’s surface. This causes possible effects on the regional climate. A change in regional climate can affect agricultural methods for farmers since crops grow more productively at particular temperatures. Thus, impacting what kinds of crops can be grown in different regions and ultimately effecting the yield farms collect per growing season. Change in regional climate can also change the diversity of life within the regions ecosystem for similar reasoning. Organisms are known to be affected by climate change. This is because organisms such as macroinvertbrates have thermal neutral zones [1]. Thermal neutral zones are the tolerance ranges of a specific species of organisms. Therefore, fluctuations and changes in climate can alter the biodiversity in a given region since climate changes may force some species to operate outside their climate tolerance. When this occurs these species may die off, therefore reducing the biodiversity in the region. However, climate change may increase the populations of endemic species or provide ecological opportunity for new species. This is because the change organisms that optimally operate at the new temperatures, due to the climate change, will thrive and reproduce. The net effect of the climate change will then be a reduction, if new species do not replace endemic species, or a change in biodiversity, if new species do replace endemic species. These phenomena demonstrate P. syringaes’ importance to agricultural methods and biodiversity on a regional scale.
Conclusion
P.syringae are an important part of Earth’s weather system and ecosystem structure. This is because P.syringae’s significant role in bioprecipitation and the water cycle. The mechanisms of their ice nucleation and cloud seeding has been proven to be a result of their IanZ protein. The IanZ protein is the driving force in P.syringae’s ice nucleation abilities. The effects P.syringae has on bioprecipation carries over to effects on the nitrogen cycle and climate change. This implications can alter the landscape of a region, thereby further impacting biodiversity in ecosystems and agricultural methods for farmers. P.syringae are an important part of the microbial community that impacts abiotic and biotic ecosystem factors in various ways.
References
Authored for BIOL 238 Microbiology, taught by Joan Slonczewski, 2016, Kenyon College.
1.Burgmer, T. et al. 2007. Effects of climate-driven temperature changes on the diversity of freshwater macroinvertebrates. Oecologia Vol. 151: 93-103.
http://download.springer.com/static/pdf/405/art%253A10.1007%252Fs00442-006-0542-9.pdf?originUrl=http%3A%2F%2Flink.springer.com%2Farticle%2F10.1007%2Fs00442-006-0542-9&token2=exp=1461612065~acl=%2Fstatic%2Fpdf%2F405%2Fart%25253A10.1007%25252Fs00442-006-0542-9.pdf%3ForiginUrl%3Dhttp%253A%252F%252Flink.springer.com%252Farticle%252F10.1007%252Fs00442-006-0542-9*~hmac=1d0eca244036a3758432a3ddd64c9a7bd8d9da38d61bc77ae9a6a025422223a7
2.Christner, Brent C. "Cloudy With a Chance of Microbes." Microbe 7.2 (2012): 70-75. Christner Research Group. Louisiana State University. Web. 28 Oct. 2012. http://brent.xner.net/pdf/Christner2012_CloudyMicrobes.pdf
3.Cid, F.P. et al. 2016. Properties and biotechnological applications of ice binding proteins in bacteria. FEMS Microbiology Letters. http://femsle.oxfordjournals.org/content/femsle/early/2016/04/15/femsle.fnw099.full.pdf
4.Erisman, J.W. 2013. Consequences of human modification of the global nitrogen cycle. Phil Trans R Soc. B 368: 20130116. http://rstb.royalsocietypublishing.org/content/royptb/368/1621/20130116.full.pdf 5. Getz, S. et al. 1983. Scanning electron microscopy of infection sites and lesion development on tomato fruit infected with pseudomonas syringae pv. tomato. Cytology and Histology Vol. 1. No. 1: 39-43. http://www.apsnet.org/publications/phytopathology/backissues/Documents/1983Articles/Phyto73n01_39.PDF
6.Graether, S.P. and Jia, Z. 2001. Modeling pseudomonas syringae ice-nucleation protein as a -helical protein. Biophysical Journal. Vol 80: 1169-1173. http://ac.els-cdn.com/S0006349501760936/1-s2.0-S0006349501760936-main.pdf?_tid=19bf5122-0837-11e6-8f07-00000aacb35d&acdnat=1461294523_f0821815d32d5e89b548f49000de9d2c
7.Kajava, A.V. and Lindow, S. E. 1993. A model for three-dimensional structure of ice nucleation proteins. J. Mol. Biol. Vol 232: 709-717. http://ac.els-cdn.com/S0022283683714245/1-s2.0-S0022283683714245-main.pdf?_tid=df0ee12a-0834-11e6-9433-00000aacb35f&acdnat=1461293565_d8dd31a5da5445e945bf0c2effb17201
8.Maki et al. 1974. Ice Nucleation induced by Pseudomonas syringae. American Society for Microbiology Vol. 28, No. 3: 456-459. http://aem.asm.org/content/28/3/456.full.pdf+html
9.Morris, C. E. et al. 2013. The life history of Pseudomonas syringae: linking agriculture to earth system processes. Annu. Rev. Phytopathol. Vol. 51: 85–104 http://www.annualreviews.org/doi/pdf/10.1146/annurev-phyto-082712-102402
10.Morris et al. 2008. The life history of the plant pathogen Pseudomonas syringae is linked to the water cycle. The ISME Journal Vol. 2: 321–334
http://www.nature.com/ismej/journal/v2/n3/full/ismej2007113a.html
11.Morris C.E., Sands D.C., Vanneste J.L., Montarry J, Oakley B, et al. 2010. Inferring the evolutionary history of the plant pathogen Pseudomonas syringae from its biogeography in headwaters of rivers in North America, Europe and New Zealand. mBio 1(3):e00107-10 http://mbio.asm.org/content/1/3/e00107-10.full.pdf+html
12.Morris et al. 2014. Bioprecipitation: a feedback cycle linking Earth history, ecosystem dynamics and land use through biological ice nucleators in the atmosphere. Global Change Biology Vol. 20: 341–351 http://onlinelibrary.wiley.com/doi/10.1111/gcb.12447/epdf
13.Parrott, Carolyn C. 1993. Recombinant DNA to Protect Crops. Woodrow Wilson Biology Institute. https://web.archive.org/web/20120918071426/http://www.woodrow.org/teachers/bi/1993/recombinant.html
14.Pesciaroli C, Cupini F, Selbmann L, Barghini P, Fenice M. 2012. Temperature preferences of bacteria isolated from seawater collected in Kandalaksha Bay,White Sea, Russia. Polar Biol. 35:435–45
http://wsbs-msu.ru/res/DictionaryAttachment/602/DOC_FILENAME/C%20Pesciaroli%20et%20al%202012%20Termperature%20preferences%20of%20bacteria%20isolated%20from%20seawater%20collected%20in%20Kandaklaksha%20Bay.pdf
15.Prasanthm M, et al. 2015. Pseudomonas syringae: An overview and its future as a “rain making bacteria”. International Research Journal of Biological Sciences Vol. 4(2): 70-77. http://www.isca.in/IJBS/Archive/v4/i2/13.ISCA-IRJBS-2014-229.pdf
16.Slonczewski, Joan L., and John Watkins. Foster. Microbiology: An Evolving Science. First ed. New York: W.W. Norton Et, 2009. Print. Chapter 22.4. Figure 22.11A. http://books.wwnorton.com/books/webad.aspx?id=4294977366
17.Wolber, P.K. et al. 1986. Identification and purification of a bacterial ice-nucleation protein. Proc. Natl. Acad. Sci. USA. Vol. 83, pp. 7256-7260. http://www.pnas.org/content/83/19/7256.full.pdf