Geomicrobiology: Difference between revisions
Calgilbert (talk | contribs) |
|||
(150 intermediate revisions by 8 users not shown) | |||
Line 1: | Line 1: | ||
{{Curated}} | |||
[[File:Desulfovibrio_desulfuricans.jpeg|300px|thumb|right|Scanning electron micrograph of <i>Desulfovibrio desulfricans</i>, a Gram-negative sulfate-reducing bacteria. [http://www.lbl.gov/Publications/Currents/Archive/Apr-30-2004.html Image Source]]]Geomicrobiology is the interdisciplinary study of the interactions of microorganisms with earth materials. It concerns the role of microbes in geological and geochemical processes. The field of geomicrobiology has revealed new insights into the intersection of life with the physical and chemical composition of Earth’s surface. Soil microbes play a large role in the transformations of elements and minerals <ref name=USGS>U.S. Geological Survey http://microbiology.usgs.gov/geomicrobiology.html</ref>. The interactions between microbes and elements and minerals is especially important in the near surface environment known as Earth’s Critical Zone, where biotic and abiotic factors regulate the conditions for life-sustaining resources. Virtually all elements can be transformed by microbes and many elemental cycles depend on soil microbes <ref name=Gadd>Gadd, G. M. 2010. Metals, minerals and microbes: geomicrobiology and bioremediation. Microbiology, 156(3), 609-643.</ref>. The relationships between microorganisms and these inorganic compounds have important implications for both the surrounding natural environment and human use. Soil microbes can be used by humans in mineral resource extraction and bioremediation. <!-- Introduction. Maybe add image here --> | |||
==Key processes of geomicrobiology== | |||
Geomicrobiological processes are relevant in many natural environments including aquifers, geological and geochemical processes, extreme environments (acidic, extreme temperatures and saline conditions) and metal ion reduction. | |||
Changes in the mobility and availability of metal is governed by redox reactions, complexation by metabolites and siderophores, and methylation. Immobilization transforms metals and other inorganic substances into microbial biomass. [http://chemwiki.ucdavis.edu/Core/Organic_Chemistry/Reactions/Oxidation_and_Reduction_Reactions Oxidation and reduction] reactions are used by microbes to gain energy during respiration and photosynthesis, resulting in the immobilization and mineralization of metals and nutrients. Redox reactions also drive the nitrogen cycle, and reduce toxic compounds <ref name=Gadd /> Conjugation reactions are another process by which toxic chemicals are converted to polar components. These conjugated substances are then made unavailable by immobilization or they become recalcitrant and accumulate in the food web <ref name=Hall>Hall , J.C., Wickenden ,J.S., Kerrm Y. F. Yau. 2009. Biochemical Conjugation of Pesticides in Plants and Microorganisms: An Overview of Similarities and Divergences. Pesticide Biotransformation in Plants and Microorganisms. 5, 89-118.</ref> | |||
===Weathering=== | |||
Weathering plays an important role on Earth ecosystems and is important for the release of nutrients into the biosphere as a result of rock dissolution or the regulation of long-term climate by the consumption of atmospheric carbon dioxide from silicate alterations. Weathering has been considered a strictly physical and chemical process. Nonetheless, many weathering processes can be affected by the presence of microbial communities. All types of rocks are susceptible to microbial weathering, including siliceous and calcareous rocks. In general microbial weathering is due to the formation of organic acids or the production of metal-chelating siderophores on the surface of rock or minerals. Other processes include oxidative or reductive conditions of metals on rocks or minerals <ref name=Herrera>Herrera, A., Cockell, C. S., Self, S., Blaxter, M., Reitner, J., Arp, G., Drose, W., Thorsteinsson, T. , Tindle, A. G. 2008. Bacterial Colonization and Weathering of Terrestrial Obsidian in Iceland. Geomicro. J. 25: 25-37.</ref>. | |||
=== | ===Precipitation of carbonates and phosphates=== | ||
= | The influence of bacteria in mineral precipitation has been described in different natural habitats including aquatic environments and terrestrial systems. Carbonate precipitation is controlled by bacterial processes and one of the mechanisms proposed is the oxidation of ammonium by ammonium oxidizers which decreases environment pH. Other authors report that the matrix of extracellular polymeric secretions (EPS) affects mineral precipitation Simultaneous precipitation of struvite (MgNH4PO4•6H2O) associated with bacterial activity has been described in vitro carbonate precipitation experiments as well as carbonate and phosphate precipitation by bacteria from saline soils <ref name=Delgado>Delgado, G. Delgado, R., Parraga, J., Rivadeneyra, M. A., Aranda, V. 2008. Precipitation of Carbonates and Phosphates by Bacteria in Extract Solutions from a Semi-arid Saline Soil. Influence of Ca2+ and Mg2+ Concentrations and Mg2+/Ca2+ Molar Ratio in Biomineralization. Geomicro. J. 25:1–13.</ref>. | ||
Microbial | ==Examples of microbial metabolism of Minerals and inorganic substances== | ||
[[File:Mineral_Metabolism.gif|thumb|300px|right|A comprehensive depiction of the effects of microbial mineral metabolism [http://mic.microbiologyresearch.org/content/journal/micro/10.1099/mic.0.037143-0 Image Source]]] | |||
{| class="wikitable" border="1" | |||
!colspan="2"|Table of Microbial Roles for Representative Elements (Taken from Gadd 2010)[2] | |||
|- style="text-align:center" | |||
|<b>Elements</b> || <b>Microbial roles in elemental cycles</b> | |||
|- | |||
|Phosphorus (P) || Dissolution of inorganic phosphate minerals in soils; Decomposition of organic phosphorus; Release of organically bound phosphorus by use of phosphatase enzymes; Assimilation and transformation of inorganic phosphorus species; Transformations of soil organic phosphorus; Phosphorus transfer to plants by mycorrhizae | |||
|- | |||
|Sulfur (S) || Degradation of organic sulfur compounds; Sulfur transformations; Assimilation of organic and inorganic sulfur; Sulfidogenesis, Sulfur accumulation, SO<sub>4</sub><sup>2-</sup> reduction and assimilation; Oxidation of H2S to S(0) and reduction of S(0) to H<sub>2</sub>S | |||
|- | |||
|Iron (Fe) || Bioweathering of iron-containing minerals in soil; Iron solubilization by siderophores; Fe(III) reduction to Fe(II); Fe(II) oxidation to Fe(III); Iron biomineralization to oxides, hydroxides, carbonates, sulfides, etc. Metal sorption to iron oxides | |||
|- | |||
|Manganese (Mn) || Mn(II) oxidation and immobilization as Mn(IV) oxides; Bioaccumulation of Manganese oxides to surfaces and exopolymers; contribution to desert varnish formation; biosorption; accumulation; intracellular precipitation; biomineralization; metal sorption to manganese oxides | |||
|- | |||
|Magnesium (Mg), Calcium (Ca), Nickel (Ni), Zinc (Zn), Cadmium (Cd), and Strontium (Sr) || Bioweathering of minerals in soil; biosorption; uptake and accumulation; bioprecipitation of oxalates, sulfides, carbonate, phosphates, etc. | |||
|- | |||
|Copper (Cu) || Mobilization from copper containing minerals in soils; CuS formation; biosorption; uptake and accumulation; bioprecipitation | |||
|- | |||
|Mercury (Hg) || Biomethylation; Reduction of Hg(III) to Hg(0); Oxidation of Hg(0) to Hg(II). Mercury volatilization as Hg(0); degradation of organomercurials; biosorption; accumulation | |||
|- | |||
|Selenium (Se) || Reductive transformation of Se (VI) to Se(IV) to Se(0); Se(0) oxidation; Biomethylation and demethylation; assimilation of organic and inorganic Se compounds | |||
|} | |||
== | ===Manganese=== | ||
In soil, Mn typically exists as bound to metal or as an oxide, and is most abundant as MnO2.[2] Of the several oxidation states Mn has in nature, only three (II, III, and IV) are biologically important. Other than as a component of enzymes in living organisms, Mn also serves as a source of energy (i.e. as an electron acceptor and donor). Furthermore, of the three biologically important oxidation states, only Mn (II) is soluble, Mn-reduction may also serve to extract mineral Mn from soil to fulfill nutritional needs <ref name=Das>Das, A. P., Sukla, L. B., Pradhan, N., & Nayak, S. 2011. Manganese biomining: a review.Bioresource technology, 102(16), 7381-7387.</ref> | |||
Mn(II)-oxidizing bacteria are a diverse group found in almost all environments. These bacteria are up to 5 orders of magnitude faster than abiotic reactions in the production of Mn oxides which have an amorphous structure with a high surface area. The mechanism of Mn(II) oxidation by these bacteria is not clear, although recent outcomes from studies with Bacillus sp. strain SG-1 have shown that a Mn(III) is an intermediate in the final oxidation of Mn(II) through enzymatic activity <ref name=Murray>Murray, K.J., Tebo, B.M. 2007. Cr(III) Is Indirectly Oxidized by the Mn(II)-Oxidizing Bacterium Bacillus sp. Strain SG-1. Environ. Sci. Technol., 41, 528-533.</ref> | |||
===Uranium-Nitrate relationship=== | |||
Uranium occurs naturally in soils, and is mined for use in energy and weapon production. Contamination of aquifers by Uranium produced from nuclear weapons and fuel is becoming a real problem in different parts of the world. These aquifers are generally oxidized, therefore uranium predominantly exists in the reactive state U(VI). The U(VI) is often coupled with carbonate making the compound quite soluble and easy to leach. | |||
Uranium reduction occurs under anaerobic conditions with Fe(III) and/or sulfate reduction. The biogeochemical processes that occur after uranium reduction are poorly understood. Recent research has shown that the addition of nitrate (a common co-contaminant with uranium) to U(IV)-containing sediments leads to the oxidation and remobilization of U(IV). Microorganisms responsible in this process are believed to be associated with nitrate dependent Fe(II)-oxidizing microorganisms <ref name=Senko>Senko, J. M., Suflita, J. M., Krumholz, L. R. 2005. Geochemical Controls on Microbial Nitrate-Dependent U(IV) Oxidation. Geomicro. J. 22:371-378.</ref> | |||
== | ===Mineral Cycles Involving Microbes=== | ||
Microbes are involved in and drive many geochemical cycles. Among them, [https://microbewiki.kenyon.edu/index.php/Nitrogen_Cycle the nitrogen cycle], the phosphorus cycle, the sulfur cycle, and the iron cycle. | |||
====Phosphorus Cycle==== | |||
[[Image:Phosphorus_Cycle.jpeg|thumb|350px|right|The Phosphorus Cycle, from a soil perspective. [https://en.wikipedia.org/wiki/File:Phosphorus_Cycle_copy.jpg Image Source]]] | |||
Phosphorus is an important nutrient for all living organisms. Most phosphorus found in living systems are in the form of inorganic phosphate. On Earth virtually all known phosphorus exists in the +5 oxidation state. Nonetheless, there are also two additional known forms: phosphonates (+3) and phosphonates (+1). Microbes immobilize, mineralize, and solubilize phosphorus <ref name=EnvLitPhosphorus>The Environmental Literacy Council. Phosphorus cycle. http://enviroliteracy.org/air-climate-weather/biogeochemical-cycles/phosphorus-cycle/</ref>. | |||
The phosphorus cycle does not include a gas phase, and thus very little phosphorus makes its way into the atmosphere (small amounts of phosphoric acid can make their way into the atmosphere to contribute to acid rain). The phosphorus cycle begins in sedimentary rock and is released into the soil and water through weathering. Microbes (and plants, as well) assist in the solubilization of mineral phosphorus in soil through the production of acids to lower the soil pH, as well as the secretion of chelating agents to bind the metal ions that would bind to the phosphate. These soluble phosphates are immobilized by living organisms, and can be produced through mineralization reactions <ref name=Sylvia>Sylvia, D. M., Hartel, P. G., Fuhrmann, J. J., Zuberer, D. A. Principles of Applications of Soil Microbiology. 2nd edition. 2005. Pearson Prentice Hall.</ref>. | |||
<i>Actinomycetes</i>, <i>Pseudomonas</i>, and <i>Bacillus</i> species are among the bacteria that solubilize inorganic phosphorus. <i>Aspergillus</i> and <i>Penicillium</i> are among the fungi that solubilize phosphorus. Additionally in the rhizosphere, mycorrhizal symbioses between fungi and plants greatly increase phosphorus uptake by plants. Some of the fungi involved are <i>Zygomycetes</i> in the order of <i>Mucorales</i> and <i>Ascomycetes</i> <ref name=Rosling />. | |||
Microbes also increase the mineralization process of phosphorous in soil by using phosphatase enzymes to hydrolyze organic phosphorus and convert it back to its inorganic form <ref name=Sylvia />. | |||
====Sulfur Cycle==== | |||
[[File:Sulfur.jpg|thumb|350px|left|The sulfur cycle. This example show sulfur cycling in the Everglades. [http://pubs.usgs.gov/fs/fs109-03/fs109-03.html Image Source]]] | |||
Most sulfur is contained in rock, with small amounts in the atmosphere and water. In soil, however, most sulfur is organic, as part of an organism; the addition of new sulfur into the ecosystem is through the weathering of sulfur-containing stone. Thus, the sulfur cycle in soil is driven by microorganisms. Some of the microbially-induced transformations sulfur undergoes are: reduction and oxidation, mineralization and immobilization, and volatilization. In short, sulfur bacteria convert mineral sulfur into soluble forms, which are then taken up into microbial biomass, or converted into other mineral forms. Sulfur exits the soil through leaching or volatilization of sulfur <ref name=Klotz>Klotz, M. G. (2011). BryantDA and Hanson TE (2011) The microbial sulfurcycle. The microbial sulfur cycle, 5.</ref>. | |||
As an elemental substance, sulfur is important in that it has a wide range of stable redox states. Inorganic sulfur can be used as a terminal electron acceptor for energy. Sulfate-reducing bacteria are comprised of several groups of bacteria that use sulfate as an oxidizing agent, reducing it to sulfide. Most sulfate-reducing bacteria can also use other oxidized sulfur compounds such as sulfite and thiosulfate, or elemental sulfur. This type of metabolism is called dissimilation, since sulfur is not incorporated or assimilated into any organic compounds <ref name=Campbell>Campbell, B. J., Engel, A. S., Porter, M. L., & Takai, K. (2006). The versatile ε-proteobacteria: key players in sulphidic habitats. Nature Reviews Microbiology, 4(6), 458-468.</ref>. | |||
Anaerobic sulfur reducing bacteria reduce sulfate ions to hydrogen sulfide. These sulfur reducing bacteria are heterotrophic organisms that use sulfur ions as terminal electron acceptors in their metabolism. These organisms include bacteria of the genera <i>Desulfovibrio</i> and <i>Desulfotomaculum</i>. | |||
There is a very | The bacterial genera <i>Thiobacillus</i>, <i>Sulfolobus</i>, and <i>Thiomicrospira</i> oxidize sulfur to produce sulfuric acid as a byproduct. These bacteria accelerate the generation of [http://technology.infomine.com/enviromine/ard/Microorganisms/roleof.htm acid rock drainage] (ARD) from pyritic and pyrrhotitic rocks. <i>Acidithiobacillus thiooxidans</i> also produces sulfuric acid and, in conjunction with others of the same genus, is currently used in a mining technique called bioleaching whereby metals are extracted from their ores through oxidation. The bacteria are used as catalysts in the oxidation reaction. Sulfate-reducing bacteria have been considered for remediating water contamination produced by other bacteria from acid mine tailings. | ||
====Iron Cycle==== | |||
[[File:Mineral_Cycle.jpg|thumb|300px|right|A representation of the general metal cycle. The iron cycle, in specific, is known as the ferrous wheel -- a cycle where Fe(III) is microbially reduced into Fe(II), which is then chemically or microbially oxidized back into Fe(III). The reduction of Fe(III) occurs through anaerobic respiration, where iron is used as a terminal electron acceptor. The oxidation of Fe(II) occurs through several pathways, and can occur in both aerobic and anaerobic conditions. [http://www.lifesci.dundee.ac.uk/people/geoff-gadd Image Source]]] | |||
After oxygen, iron is the most abundant redox-active element in the Earth’s crust. However, it is not easily available in its preferred state <ref name=Emerson>Emerson, D., Roden, E., Twining, B.S. 2012. The microbial ferrous wheel: iron cycling in terrestrial, freshwater, and marine environments. Frontiers in Microbiolog</ref>. The availability of iron depends on the aerobic condition and pH level of the soil. Under aerobic conditions, iron readily binds with oxygen, forming insoluble (and therefore non-bioavailable) iron oxides. However, at low pH, both common forms of Fe (II, III) are readily soluble. In terms of mineralization and solubilization, microbes accomplish both through redox reactions <ref name=Colombo>Colombo, C., Palumbo, G., He, J. Z., Pinton, R., & Cesco, S. (2014). Review on iron availability in soil: interaction of Fe minerals, plants, and microbes.Journal of soils and sediments, 14(3), 538-548.</ref>. Iron can be mineralized, immobilized, oxidized, and reduced. | |||
Fe(III) is an important electron acceptor in organic matter decomposition in saturated soil. As this form of iron is soluble, the iron leaves the soil; this causes gleying of the soil (the color of the soil becomes grey or blue), as the color of the sand’s sand content becomes dominant. Fe(III) reduction also corrodes steel which causes many industrial problems. One of the most common iron redox reactions in anaerobic soils is the mineralization of iron through binding sulfur to iron, producing pyrite <ref name=Colombo />. Furthermore, in these flooded conditions, iron is also used as an energy source; anaerobic iron-reducing bacteria use iron as an terminal electron acceptor in respiration <ref name=Lovley>Lovley, D. R., Holmes, D. E., & Nevin, K. P. (2004). Dissimilatory fe (iii) and mn (iv) reduction.Advances in microbial physiology, 49, 219-286.</ref>. | |||
Siderophores are iron transporting ligands that are produced by bacteria and fungi that reduce Fe(III) to Fe(II). When microbes experience iron deficiency, siderophores are secreted into the environment to capture iron. The solubilized iron is then taken up by the microbe through a siderophore receptor protein on the outer cell membrane. Iron is the only known essential element for which these specific organic shuttles operate. | |||
==Examples of Geomicrobiology Interactions in nature== | |||
===Rhizosphere=== | |||
In terms of geobiology, microbes in the rhizosphere serve to alter soil concentration of metals and other minerals In terms of geobiology, microbes in the rhizosphere serve to alter soil concentration of metals and other minerals to assist plants in a symbiotic manner, both indirectly and directly. Low concentrations of bioavailable minerals activate and stimulate the activity of these microorganisms in these biogeochemical processes. Microbes capable of generating these processes, altering the mineral concentration and composition of the rhizosphere soil, include those part of [https://microbewiki.kenyon.edu/index.php/Nitrogen_Cycle the nitrogen cycle], siderophore-producing microbes, and phosphorus-solubilizing microorganisms. | |||
Iron, as an element, is relatively abundant in soil; however, most of that iron is sequestered in various inorganic iron compounds inaccessible to both plants and microbes <ref name="Colombo" />. Iron deficiency stimulates the production and secretion of siderophores <ref name="Saha">Saha, R., Saha, N., Donofrio, R. S., & Bestervelt, L. L. (2013). Microbial siderophores: a mini review. Journal of basic microbiology, 53(4), 303-317.</ref>. Microbial siderophores are chelating agents (organic compounds that can bind to metal ions) secreted by bacteria and fungi, used to capture free iron ions in the soil and, as highly soluble substances, transport that iron to the microorganisms. More importantly from a geochemical point of view, siderophores can also increase the concentration of iron ions in the soil through stimulating the release of inorganic iron (usually from iron oxide crystals, which trap most of the inaccessible iron in the soil). There are three general categories of methods used to promote this release: direct reaction at the surface of the iron oxide crystal; ligand-promoted exchange of iron ions; and the protonation of coordinating partners of iron ions to free the ions <ref name=Kraemer>Kraemer, S. M. (2004). Iron oxide dissolution and solubility in the presence of siderophores. Aquatic sciences, 66(1), 3-18.</ref>. | |||
Inorganic phosphorus in soil is usually complexed with metal ions, a reaction that happens very fast. For example, the spike in concentration of soluble (bioavailable) phosphorus from addition of chemical fertilizers decreases to normal levels in a few days <ref name="Sindhu">Sindhu, S. S., Phour, M., Choudhary, S. R., & Chaudhary, D. (2014). Phosphorus Cycling: prospects of using rhizosphere microorganisms for improving phosphorus nutrition of plants. In Geomicrobiology and biogeochemistry (pp. 199-237). Springer Berlin Heidelberg.</ref>. This complexation is dependent on many factors, among them, the pH of the soil. Thus, the commonly accepted method for microbial-caused dissolution of phosphorus is through the activity of low molecular weight (i.e. highly soluble) organic acids <ref name=Khan>Khan, M. S., Zaidi, A., & Ahmad, E. (2014). Mechanism of phosphate solubilization and physiological functions of phosphate-solubilizing microorganisms. In Phosphate Solubilizing Microorganisms (pp. 31-62). Springer International Publishing.</ref>. Though the exact mechanisms are still unknown, the accepted theory is that carboxylic acids act as chelating agents for the metal ions complexed with phosphorus, preventing precipitation of the free phosphorus ions released with a drop of pH <ref name=Sindhu />. That is, the metal phosphorus complexes were disrupted by a drop in pH induced by the organic acids, the carboxylic acids caught the released metal ions, and therefore phosphorus ions was released into solution, where plants and microbes can uptake the phosphorus. | |||
====Volcanic Hot Springs==== | |||
[[File:Sulfur_Rocks.jpg|thumb|200px|right| Sulfur deposits by microbes at a hot spring. [http://www.lpi.usra.edu/science/treiman/greatdesert/workshop/sulphursprings/ Image Source]]] | |||
Thermophilic Microbes occur in volcanic hot springs around the world. Sulfur is an element commonly found in and around hot springs. The spring water is rich in sulfides that leach out of the rocks and soil, which is then oxidized by the microbes. The microbes strip electrons from the sulfide to use in their metabolic pathways, leaving behind sulfur. Other kinds of bacteria can oxidize sulfide or sulfur to sulfate, and form sulfuric acid. This is why many hot springs are acidic. | |||
Different kinds of metabolic lifestyles can be found in different volcanic environments. For example, in the Kamchatka Hot Springs, lithotrophic methanogenesis was found to occur at alkaline conditions with a neutral pH. Sulfidogenesis occurred at neutral and acidic pH, and lithotrophic acetogenesis occurred over the entire range of pH. Lithotrophic reduction of Fe(III) also occurred over the entire range of pH. In these conditions, the only source of metabolic substrate comes from volcanic gases and methanogenesis is the process most commonly associated with hot spring microbial activity <ref name="Bonch">Bonch-Osmolovskaya, E. A., Miroshnichenko, M. L., Slobodkin, A. I., Sokolova, T. G., Karpov, G. A., Kostrikina, N. A., ... & Pimenov, N. V. (1999). Biodiversity of anaerobic lithotrophic prokaryotes in terrestrial hot springs of Kamchatka. MICROBIOLOGY-AIBS-C/C OF MIKROBIOLOGIIA, 68, 343-351.</ref>. | |||
==Examples of Applied Geomicrobiology== | |||
Geomicrobiology has many useful applications for humans. Many microbes are involved with breaking down toxic compounds (see [https://microbewiki.kenyon.edu/index.php/Bioremediation Bioremediation]), mineral extraction, and the manufacturing of valuable minerals and compounds. | |||
===Space exploration=== | |||
Geomicrobiology is being considered an important tool in the exploration and settlement of space because microorganisms can be used for a variety of uses, such as life support and waste management, energy production, extraction of industrially useful minerals, and the search for life using microbe-mineral interactions. Below are a couple of examples of how humans can use processes that are studied on earth to help explore and colonize space. | |||
====Life Support and Waste Management==== | |||
[[Image:Melissaloop.jpeg|thumb|300px|right|General concept of the MELISSA loop for advanced life support [http://ecls.esa.int/ecls/?p=newmelissaloop Image Source]]] | |||
The biggest use of microbes in space exploration and settlement would be the use of photosynthetic organisms to convert water and carbon dioxide into oxygen and food in what is known as a biological life support system. Additionally the waste from these processes could be used to make more carbon dioxide, recycling the nutrients, water and carbon. The Micro Ecological Life Support System Alternative (MELiSSA), designed by European Space Agency, is a model system of an advance biological life support system that would be based on different species of microbes and plants. Consisting of many bioreactors and plant chambers, MELiSSA would break down the organic waste from the crew and non-edible parts of the higher plants <ref name="Godia">Godia, F., Albiol, J., Montesinos, J. L., Pérez, J., Creus, N., Cabello, F., ... & Lasseur, C. (2002). MELISSA: a loop of interconnected bioreactors to develop life support in space. Journal of biotechnology, 99(3), 319-330.</ref>. We can learn a lot about how MELiSSA works by looking at soil systems and processes here on Earth. | |||
The MELiSSA loop is the basis of the life support system created by the ESA and uses processes we see all the time on earth. For example, the transformations of nitrogen (mineralization, nitrification, immobilization and denitrification) show the different ways nitrogen is transformed in soil and in the atmosphere. From studying how microbes interact in soil has helped us create MELiSSA, which combines multiple systems to produce food, water and oxygen for space explorers. | |||
====Energy Production==== | |||
One example of the use of microbes in in the production of energy and additional waste management is the use of a MFC, or microbial fuel cell. MFCs can be used to generate electricity and process waste, but they currently produce low amounts of energy and depend on microbes processing organic matter in an anaerobic anode chamber <ref name=Cockell>Cockell, C. S. (2010). Geomicrobiology beyond Earth: microbe–mineral interactions in space exploration and settlement. Trends in microbiology, 18(7), 308-314.</ref>. On earth MFCs can be made using the soil found all around us because soil contains a diverse population of microbes, along with the nutrients needed by those microbes to generate energy. | |||
===Microbial Mineral Resource Extraction=== | |||
====Gold Production, Detection, and Transformation by Microbes==== | |||
[[File:Gold_bacteria.jpg|thumb|150px|left|<i>Cupriavidus metallidurans</i> bacteria metabolize toxic gold chloride into gold nanoparticles (white). Photo from Frank Reith and caption from Gwynne. [http://www.nature.com/nature/journal/v495/n7440_supp/full/495S12a.html Image Source]]] | |||
Some bacteria are capable of reducing toxic gold chloride into pure gold. Cupriavidus metallidurans is one of the few bacteria that can survive in high concentrations of heavy metal in mines throughout the world <ref name=Gywnne>Gwynne, P. (2013). Microbiology: There's gold in them there bugs. Nature, 495(7440), S12-S13.</ref>. Although the bacteria only produce small amounts of gold nanoparticles too small to be profitable, they can be used by miners as biosensors to detect the concentrations of gold in environmental samples. Additionally, the gold nanoparticles synthesized by the bacteria could have potential in applications for many kinds of technology, such as optoelectronics, imaging technology, and drug delivery. Transcriptomic microarrays and DNA sequencing can detect bacterial genes that are associated with gold transformation, and thus pinpoint where significant gold deposits are present. There is great interest for the application of microbes in transforming toxic salts into gold, and scientists are studying both marine and terrestrial species to look for new insights into this area of geomicrobiology. | |||
====Role of Geomicrobiology in Bioleaching==== | |||
Bioleaching can use microbes to extract metal from ore and remove metal impurities without the use of cyanide, mercury, or other chemicals. Biological forms of extraction can be cleaner than chemical methods because less toxins are released by the process, and detoxification can occur on site during processing. Chemolithotrophic bacteria reduce metal sulfides to metal sulfates, and heterotrophic bacteria or fungi exude organic acids, chelating, and complexing compounds to extract other ores and minerals. Although chemical extraction is still used when it is cheaper or another process is unknown, the field of bioleaching has high potential for further development. | |||
The most active bacteria used in bioleaching are from genus Thiobacillus, gram negative bacteria that perform aerobic oxidation of sulfur compounds in acidic conditions. Other bioleaching genus’ include iron- oxidizing Leptospirillum and Thermophilic bacteria <ref name=Bosecker>Bosecker, Klaus (1997). Bioleaching: metal solubilization by microorganisms. FEMS Microbiology Review 20 (pp. 591-604)</ref>. | |||
===Role of Geomicrobiology in Removal and Disposal of Heavy Metals and Pollutants=== | |||
[[File:Cr_Spill_Durango.jpg|thumb|right|300px|A plume from a mine waste spill in Durango, Colorado, with high Cromium (VI) content has spread to northwestern New Mexico, carried on the San Juan river (Jerry McBride, The Durango Herald, AP, 2015)]] | |||
Geomicrobiology can be applied to [https://microbewiki.kenyon.edu/index.php/Bioremediation soil bioremediation], watershed reclamation, and to remove plumes of industrial contamination or radiation from the environment. Microbes in the soil and watertable are able to degrade contaminants that have no other practical source of removal and may be toxic to plants and other organisms. Inoculation of multiple target microbes can be used to remove multi-chemical pollutants from a site simultaneously<ref name=Sobolewski>Sobolewski, A. 2006. A Review of Processes Responsible for Metal Removal in Wetlands Treating Contaminated Mine Drainage, International Journal of Phytoremediation, 1:1, 19-51.</ref>. Selection and application of species must take into consideration the reactions that will take place at each step, to ensure the target compound is cleaned from the soil; for example, the desired reactions may require anaerobic or aerobic conditions in the soil and thus the introduced microbes must survive and thrive in such conditions. | |||
Extraction processes bring heavy metals and other deep subsurface compounds to the surface soil or to the watershed resulting in contamination. Processing metal ore and other resources for production with heavy metals, like mercury, also contribute to the pollution. Microbes found within these layers have evolved to breakdown, reduce, or remove these compounds in their natural settings to extract energy and nutrients through use of precipitation, redox, sorption, and metabolic processes. Target microbes are inoculated at contaminated sites and/or the conditions are changed to select for the desired native microbial community that will transform the pollutant<ref name=Parmar />. Heavy metals including arsenic, zinc and sulfur can be precipitated out of acid mine wastewaters by sulfate-reducing bacteria, producing highly insoluble metal sulfides that are retained by wetland sediments<ref name=Sobolewski />. | |||
Bacteria can reduce heavy metals from the soil in the rhizosphere for plant uptake and removal<ref name=Dimitroula>Dimitroula, H., Syranidou, E., Manousaki, E., Nikolaidis, N. P., Karatzas, G. P., & Kalogerakis, N. (2015). Mitigation measures for chromium-VI contaminated groundwater–The role of endophytic bacteria in rhizofiltration. Journal of hazardous materials, 281, 114-120.</ref>. Toxins can be converted to nontoxic forms and remain in the soil or be removed by translocation from the roots up into the plant, which can then be harvested to remove the metal from the site. For example, by providing anoxic conditions, high concentrations of toxic chromium (VI) are reduced by rhizospheric bacteria Arthrobacter and Pseudomonas to form immobile, nontoxic Cr(III)<ref name=Gutierrez>Gutiérrez, A. M., Cabriales, J. J. P., & Vega, M. M. (2010). Isolation and characterization of hexavalent chromium-reducing rhizospheric bacteria from a wetland. International journal of phytoremediation, 12(4), 317-334.</ref>. Chromium can be reduced from toxic Cr(VI) to nontoxic Cr(III) by endophytic bacteria within their cells; these Cr compounds are absorbed into the plant for easy removal from the soil and groundwater through harvesting. Some examples of endophytic bacteria species capable of Cr reduction include Ralstonia pickettii and Ochrobactrum intermedium (both gram -), and other non-endophytic examples of Cr-reducing bacteria include Bacillus cereus and Streptomyces (both gram +)<ref name=Dimitroula />. | |||
===Role of Geomicrobiology in Radiation Contaminated Soils and Groundwater=== | |||
[https://microbewiki.kenyon.edu/index.php/Radiotrophic_Fungi Radiotrophic fungi] can survive and colonize water and substrates (i.e. concrete barriers) in sites contaminated by severe radioactivity by using melanin pigment to transfer electrons and gain energy from the radioactive material. More study is needed to confirm if the radioactive material is then transformed to lower energy, safer products as the extra electrons are transferred by reduction. The fungal hyphae also deteriorate the concrete barriers by colonization and mineral weathering processes, which could result in release of radioactive material. A famous case is Chernobyl: after ten years of the catastrophe of Chernobyl in 1986 extensive fungal growth was observed on the walls and other building structures constructed in the inner part of the “Shelter” built over the fourth Unit of the Chernobyl nuclear power plant. Safe long-term storage of both existing and future nuclear waste is of vital importance in protecting the environment, therefore the study of these fungi has been key for future construction of nuclear waste repositories. Some of the microorganisms isolated from these extreme environments include modified strains from the genera Alternaria, Cladosporium, and Aureobasidium<ref name=Formina>Fomina, M., Podgorsky, V. S., Olishevska, V., Kadoshnikov, V. M., Pisanska, I. R., Hillier, S., Gadd, G. M. 2007. Fungal Deterioration of Barrier Concrete used in Nuclear Waste Disposal. Geomicro. J. 24:643-653.</ref>. | |||
===Role of Geomicrobiology in Agriculture=== | |||
As agriculture enters the 21st century, new innovations are being discovered using microbes to increase production, yield, and sustainability in cropping systems. Soil quality and conservation are also becoming priorities on the agricultural forefront to combat the unintended consequences of conventional cropping systems. Metal and sulfate-reducing bacteria are responsible for precipitation and immobilization of metals like iron or manganese, and sulfate in the soil, determining the availability of these plant limiting nutrients<ref name=Parmar>N. Parmar and A. Singh (eds.), Geomicrobiology and Biogeochemistry, Soil Biology 39, DOI 10.1007/978-3-642-41837-2_11. Springer-Verlag Berlin Heidelberg 2014</ref>. | |||
For example, Phosphate solubilizing microorganisms (PSMs) use organic acids to dissociate phosphorus in small amounts from metal-phosphate compounds, making it available for plant uptake. PSM activity can result in reduced need for P fertilizer use and is being researched for use in biofertilizers. Some examples of PSMs include Chromobacterium, Azotobacter, and Bradyrhizobium<ref name=Parmar />. Rhizobia have been used for some time to help increase Nitrogen fixation in soils, however increasing knowledge of microbial control of nutrient availability are broadening sustainable approaches to improved crop and soil health and quality. | |||
Microorganisms can be used in integrated pest management systems to fend off pests. The bacterium Thiobacillus, a genus of 'Hydrogenophilaceae', are thermophilic Proteobacteria growing in temperatures up to 50 °C. They obtain their energy from hydrogen oxidation and are used as pest control in potato fields to control scabs<ref name="Rosling">Rosling, A., Suttle, K B., Johansson, E., Van Hees, P. A. W., Banfield, J. F. 2007. Phosphorous availability influences the dissolution of apatite by soil fungi. Geobiology 5 (3): 265–280. | |||
</ref>. Microbes are also used in less toxic insecticides called [http://www.ipm.ucdavis.edu/QT/lesstoxicinsecticidescard.html Microbials]. | |||
==Current Research== | |||
===Magnetotactic Bacteria=== | |||
Magnetotactic bacteria produce small magnetite crystals to form microbial compasses. These materials are used in building microchips and electronics. | |||
For example, a number of magnetic nanomaterials are being synthesized with biomimetic techniques, which were inspired by the mineralization processes of iron these magnetotactic bacteria did, and are used in, to name a few, "high-density data storage and ferrofluidic devices, electromagnetic shielding, spintronics, and quantum computing, and applications in diagnostic medicine and targeted drug delivery"<ref name=Prozorov>Prozorov, T., Bazylinski, D. A., Mallapragada, S. K., & Prozorov, R. (2013). Novel magnetic nanomaterials inspired by magnetotactic bacteria: Topical review. Materials Science and Engineering: R: Reports, 74(5), 133-172.</ref>. | |||
===Advances in Removal of Heavy Metals and Radioactivity=== | |||
Research of microbial activities using radioactive and toxic metal waste in bedrock groundwater for safe long-term disposal is showing great potential for nuclear waste management. Corrosion of steel, copper, and other compounds is induced deep within the bedrock at greater rates than abiotic processes. The groundwater microbes bind the metal surfaces, forming biofilms resulting in corrosion of the metals<ref name=Carpen>L. Carpén, P. Rajala, M. Bomberg, "Microbially Induced Corrosion in Deep Bedrock", Advanced Materials Research, Vol. 1130, pp. 75-78, Nov. 2015</ref>. | |||
==References== | ==References== | ||
<references /> | |||
[ | Edited by students of [mailto:kmscow@ucdavis.edu Kate Scow] | ||
<!-- Do not edit or remove this line --> [[Category:Pages edited by students of Kate Scow at UC Davis]] |
Latest revision as of 01:39, 22 March 2016

Geomicrobiology is the interdisciplinary study of the interactions of microorganisms with earth materials. It concerns the role of microbes in geological and geochemical processes. The field of geomicrobiology has revealed new insights into the intersection of life with the physical and chemical composition of Earth’s surface. Soil microbes play a large role in the transformations of elements and minerals [1]. The interactions between microbes and elements and minerals is especially important in the near surface environment known as Earth’s Critical Zone, where biotic and abiotic factors regulate the conditions for life-sustaining resources. Virtually all elements can be transformed by microbes and many elemental cycles depend on soil microbes [2]. The relationships between microorganisms and these inorganic compounds have important implications for both the surrounding natural environment and human use. Soil microbes can be used by humans in mineral resource extraction and bioremediation.
Key processes of geomicrobiology
Geomicrobiological processes are relevant in many natural environments including aquifers, geological and geochemical processes, extreme environments (acidic, extreme temperatures and saline conditions) and metal ion reduction.
Changes in the mobility and availability of metal is governed by redox reactions, complexation by metabolites and siderophores, and methylation. Immobilization transforms metals and other inorganic substances into microbial biomass. Oxidation and reduction reactions are used by microbes to gain energy during respiration and photosynthesis, resulting in the immobilization and mineralization of metals and nutrients. Redox reactions also drive the nitrogen cycle, and reduce toxic compounds [2] Conjugation reactions are another process by which toxic chemicals are converted to polar components. These conjugated substances are then made unavailable by immobilization or they become recalcitrant and accumulate in the food web [3]
Weathering
Weathering plays an important role on Earth ecosystems and is important for the release of nutrients into the biosphere as a result of rock dissolution or the regulation of long-term climate by the consumption of atmospheric carbon dioxide from silicate alterations. Weathering has been considered a strictly physical and chemical process. Nonetheless, many weathering processes can be affected by the presence of microbial communities. All types of rocks are susceptible to microbial weathering, including siliceous and calcareous rocks. In general microbial weathering is due to the formation of organic acids or the production of metal-chelating siderophores on the surface of rock or minerals. Other processes include oxidative or reductive conditions of metals on rocks or minerals [4].
Precipitation of carbonates and phosphates
The influence of bacteria in mineral precipitation has been described in different natural habitats including aquatic environments and terrestrial systems. Carbonate precipitation is controlled by bacterial processes and one of the mechanisms proposed is the oxidation of ammonium by ammonium oxidizers which decreases environment pH. Other authors report that the matrix of extracellular polymeric secretions (EPS) affects mineral precipitation Simultaneous precipitation of struvite (MgNH4PO4•6H2O) associated with bacterial activity has been described in vitro carbonate precipitation experiments as well as carbonate and phosphate precipitation by bacteria from saline soils [5].
Examples of microbial metabolism of Minerals and inorganic substances
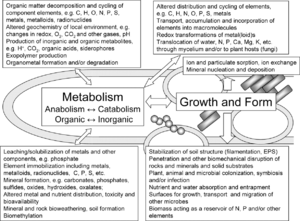
Table of Microbial Roles for Representative Elements (Taken from Gadd 2010)[2] | |
---|---|
Elements | Microbial roles in elemental cycles |
Phosphorus (P) | Dissolution of inorganic phosphate minerals in soils; Decomposition of organic phosphorus; Release of organically bound phosphorus by use of phosphatase enzymes; Assimilation and transformation of inorganic phosphorus species; Transformations of soil organic phosphorus; Phosphorus transfer to plants by mycorrhizae |
Sulfur (S) | Degradation of organic sulfur compounds; Sulfur transformations; Assimilation of organic and inorganic sulfur; Sulfidogenesis, Sulfur accumulation, SO42- reduction and assimilation; Oxidation of H2S to S(0) and reduction of S(0) to H2S |
Iron (Fe) | Bioweathering of iron-containing minerals in soil; Iron solubilization by siderophores; Fe(III) reduction to Fe(II); Fe(II) oxidation to Fe(III); Iron biomineralization to oxides, hydroxides, carbonates, sulfides, etc. Metal sorption to iron oxides |
Manganese (Mn) | Mn(II) oxidation and immobilization as Mn(IV) oxides; Bioaccumulation of Manganese oxides to surfaces and exopolymers; contribution to desert varnish formation; biosorption; accumulation; intracellular precipitation; biomineralization; metal sorption to manganese oxides |
Magnesium (Mg), Calcium (Ca), Nickel (Ni), Zinc (Zn), Cadmium (Cd), and Strontium (Sr) | Bioweathering of minerals in soil; biosorption; uptake and accumulation; bioprecipitation of oxalates, sulfides, carbonate, phosphates, etc. |
Copper (Cu) | Mobilization from copper containing minerals in soils; CuS formation; biosorption; uptake and accumulation; bioprecipitation |
Mercury (Hg) | Biomethylation; Reduction of Hg(III) to Hg(0); Oxidation of Hg(0) to Hg(II). Mercury volatilization as Hg(0); degradation of organomercurials; biosorption; accumulation |
Selenium (Se) | Reductive transformation of Se (VI) to Se(IV) to Se(0); Se(0) oxidation; Biomethylation and demethylation; assimilation of organic and inorganic Se compounds |
Manganese
In soil, Mn typically exists as bound to metal or as an oxide, and is most abundant as MnO2.[2] Of the several oxidation states Mn has in nature, only three (II, III, and IV) are biologically important. Other than as a component of enzymes in living organisms, Mn also serves as a source of energy (i.e. as an electron acceptor and donor). Furthermore, of the three biologically important oxidation states, only Mn (II) is soluble, Mn-reduction may also serve to extract mineral Mn from soil to fulfill nutritional needs [6]
Mn(II)-oxidizing bacteria are a diverse group found in almost all environments. These bacteria are up to 5 orders of magnitude faster than abiotic reactions in the production of Mn oxides which have an amorphous structure with a high surface area. The mechanism of Mn(II) oxidation by these bacteria is not clear, although recent outcomes from studies with Bacillus sp. strain SG-1 have shown that a Mn(III) is an intermediate in the final oxidation of Mn(II) through enzymatic activity [7]
Uranium-Nitrate relationship
Uranium occurs naturally in soils, and is mined for use in energy and weapon production. Contamination of aquifers by Uranium produced from nuclear weapons and fuel is becoming a real problem in different parts of the world. These aquifers are generally oxidized, therefore uranium predominantly exists in the reactive state U(VI). The U(VI) is often coupled with carbonate making the compound quite soluble and easy to leach. Uranium reduction occurs under anaerobic conditions with Fe(III) and/or sulfate reduction. The biogeochemical processes that occur after uranium reduction are poorly understood. Recent research has shown that the addition of nitrate (a common co-contaminant with uranium) to U(IV)-containing sediments leads to the oxidation and remobilization of U(IV). Microorganisms responsible in this process are believed to be associated with nitrate dependent Fe(II)-oxidizing microorganisms [8]
Mineral Cycles Involving Microbes
Microbes are involved in and drive many geochemical cycles. Among them, the nitrogen cycle, the phosphorus cycle, the sulfur cycle, and the iron cycle.
Phosphorus Cycle
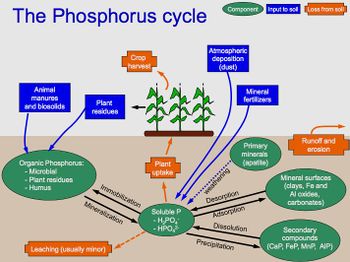
Phosphorus is an important nutrient for all living organisms. Most phosphorus found in living systems are in the form of inorganic phosphate. On Earth virtually all known phosphorus exists in the +5 oxidation state. Nonetheless, there are also two additional known forms: phosphonates (+3) and phosphonates (+1). Microbes immobilize, mineralize, and solubilize phosphorus [9].
The phosphorus cycle does not include a gas phase, and thus very little phosphorus makes its way into the atmosphere (small amounts of phosphoric acid can make their way into the atmosphere to contribute to acid rain). The phosphorus cycle begins in sedimentary rock and is released into the soil and water through weathering. Microbes (and plants, as well) assist in the solubilization of mineral phosphorus in soil through the production of acids to lower the soil pH, as well as the secretion of chelating agents to bind the metal ions that would bind to the phosphate. These soluble phosphates are immobilized by living organisms, and can be produced through mineralization reactions [10].
Actinomycetes, Pseudomonas, and Bacillus species are among the bacteria that solubilize inorganic phosphorus. Aspergillus and Penicillium are among the fungi that solubilize phosphorus. Additionally in the rhizosphere, mycorrhizal symbioses between fungi and plants greatly increase phosphorus uptake by plants. Some of the fungi involved are Zygomycetes in the order of Mucorales and Ascomycetes [11].
Microbes also increase the mineralization process of phosphorous in soil by using phosphatase enzymes to hydrolyze organic phosphorus and convert it back to its inorganic form [10].
Sulfur Cycle
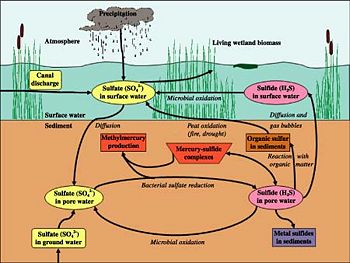
Most sulfur is contained in rock, with small amounts in the atmosphere and water. In soil, however, most sulfur is organic, as part of an organism; the addition of new sulfur into the ecosystem is through the weathering of sulfur-containing stone. Thus, the sulfur cycle in soil is driven by microorganisms. Some of the microbially-induced transformations sulfur undergoes are: reduction and oxidation, mineralization and immobilization, and volatilization. In short, sulfur bacteria convert mineral sulfur into soluble forms, which are then taken up into microbial biomass, or converted into other mineral forms. Sulfur exits the soil through leaching or volatilization of sulfur [12].
As an elemental substance, sulfur is important in that it has a wide range of stable redox states. Inorganic sulfur can be used as a terminal electron acceptor for energy. Sulfate-reducing bacteria are comprised of several groups of bacteria that use sulfate as an oxidizing agent, reducing it to sulfide. Most sulfate-reducing bacteria can also use other oxidized sulfur compounds such as sulfite and thiosulfate, or elemental sulfur. This type of metabolism is called dissimilation, since sulfur is not incorporated or assimilated into any organic compounds [13].
Anaerobic sulfur reducing bacteria reduce sulfate ions to hydrogen sulfide. These sulfur reducing bacteria are heterotrophic organisms that use sulfur ions as terminal electron acceptors in their metabolism. These organisms include bacteria of the genera Desulfovibrio and Desulfotomaculum.
The bacterial genera Thiobacillus, Sulfolobus, and Thiomicrospira oxidize sulfur to produce sulfuric acid as a byproduct. These bacteria accelerate the generation of acid rock drainage (ARD) from pyritic and pyrrhotitic rocks. Acidithiobacillus thiooxidans also produces sulfuric acid and, in conjunction with others of the same genus, is currently used in a mining technique called bioleaching whereby metals are extracted from their ores through oxidation. The bacteria are used as catalysts in the oxidation reaction. Sulfate-reducing bacteria have been considered for remediating water contamination produced by other bacteria from acid mine tailings.
Iron Cycle
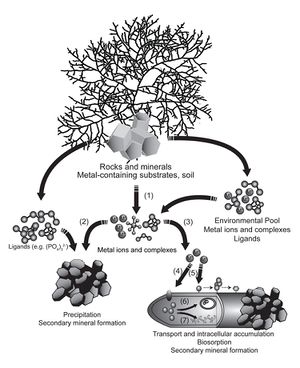
After oxygen, iron is the most abundant redox-active element in the Earth’s crust. However, it is not easily available in its preferred state [14]. The availability of iron depends on the aerobic condition and pH level of the soil. Under aerobic conditions, iron readily binds with oxygen, forming insoluble (and therefore non-bioavailable) iron oxides. However, at low pH, both common forms of Fe (II, III) are readily soluble. In terms of mineralization and solubilization, microbes accomplish both through redox reactions [15]. Iron can be mineralized, immobilized, oxidized, and reduced.
Fe(III) is an important electron acceptor in organic matter decomposition in saturated soil. As this form of iron is soluble, the iron leaves the soil; this causes gleying of the soil (the color of the soil becomes grey or blue), as the color of the sand’s sand content becomes dominant. Fe(III) reduction also corrodes steel which causes many industrial problems. One of the most common iron redox reactions in anaerobic soils is the mineralization of iron through binding sulfur to iron, producing pyrite [15]. Furthermore, in these flooded conditions, iron is also used as an energy source; anaerobic iron-reducing bacteria use iron as an terminal electron acceptor in respiration [16].
Siderophores are iron transporting ligands that are produced by bacteria and fungi that reduce Fe(III) to Fe(II). When microbes experience iron deficiency, siderophores are secreted into the environment to capture iron. The solubilized iron is then taken up by the microbe through a siderophore receptor protein on the outer cell membrane. Iron is the only known essential element for which these specific organic shuttles operate.
Examples of Geomicrobiology Interactions in nature
Rhizosphere
In terms of geobiology, microbes in the rhizosphere serve to alter soil concentration of metals and other minerals In terms of geobiology, microbes in the rhizosphere serve to alter soil concentration of metals and other minerals to assist plants in a symbiotic manner, both indirectly and directly. Low concentrations of bioavailable minerals activate and stimulate the activity of these microorganisms in these biogeochemical processes. Microbes capable of generating these processes, altering the mineral concentration and composition of the rhizosphere soil, include those part of the nitrogen cycle, siderophore-producing microbes, and phosphorus-solubilizing microorganisms.
Iron, as an element, is relatively abundant in soil; however, most of that iron is sequestered in various inorganic iron compounds inaccessible to both plants and microbes [15]. Iron deficiency stimulates the production and secretion of siderophores [17]. Microbial siderophores are chelating agents (organic compounds that can bind to metal ions) secreted by bacteria and fungi, used to capture free iron ions in the soil and, as highly soluble substances, transport that iron to the microorganisms. More importantly from a geochemical point of view, siderophores can also increase the concentration of iron ions in the soil through stimulating the release of inorganic iron (usually from iron oxide crystals, which trap most of the inaccessible iron in the soil). There are three general categories of methods used to promote this release: direct reaction at the surface of the iron oxide crystal; ligand-promoted exchange of iron ions; and the protonation of coordinating partners of iron ions to free the ions [18].
Inorganic phosphorus in soil is usually complexed with metal ions, a reaction that happens very fast. For example, the spike in concentration of soluble (bioavailable) phosphorus from addition of chemical fertilizers decreases to normal levels in a few days [19]. This complexation is dependent on many factors, among them, the pH of the soil. Thus, the commonly accepted method for microbial-caused dissolution of phosphorus is through the activity of low molecular weight (i.e. highly soluble) organic acids [20]. Though the exact mechanisms are still unknown, the accepted theory is that carboxylic acids act as chelating agents for the metal ions complexed with phosphorus, preventing precipitation of the free phosphorus ions released with a drop of pH [19]. That is, the metal phosphorus complexes were disrupted by a drop in pH induced by the organic acids, the carboxylic acids caught the released metal ions, and therefore phosphorus ions was released into solution, where plants and microbes can uptake the phosphorus.
Volcanic Hot Springs
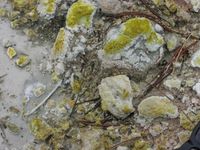
Thermophilic Microbes occur in volcanic hot springs around the world. Sulfur is an element commonly found in and around hot springs. The spring water is rich in sulfides that leach out of the rocks and soil, which is then oxidized by the microbes. The microbes strip electrons from the sulfide to use in their metabolic pathways, leaving behind sulfur. Other kinds of bacteria can oxidize sulfide or sulfur to sulfate, and form sulfuric acid. This is why many hot springs are acidic.
Different kinds of metabolic lifestyles can be found in different volcanic environments. For example, in the Kamchatka Hot Springs, lithotrophic methanogenesis was found to occur at alkaline conditions with a neutral pH. Sulfidogenesis occurred at neutral and acidic pH, and lithotrophic acetogenesis occurred over the entire range of pH. Lithotrophic reduction of Fe(III) also occurred over the entire range of pH. In these conditions, the only source of metabolic substrate comes from volcanic gases and methanogenesis is the process most commonly associated with hot spring microbial activity [21].
Examples of Applied Geomicrobiology
Geomicrobiology has many useful applications for humans. Many microbes are involved with breaking down toxic compounds (see Bioremediation), mineral extraction, and the manufacturing of valuable minerals and compounds.
Space exploration
Geomicrobiology is being considered an important tool in the exploration and settlement of space because microorganisms can be used for a variety of uses, such as life support and waste management, energy production, extraction of industrially useful minerals, and the search for life using microbe-mineral interactions. Below are a couple of examples of how humans can use processes that are studied on earth to help explore and colonize space.
Life Support and Waste Management
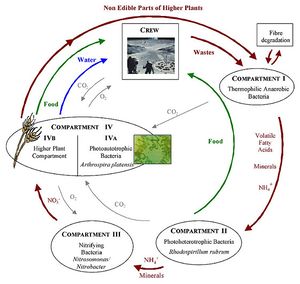
The biggest use of microbes in space exploration and settlement would be the use of photosynthetic organisms to convert water and carbon dioxide into oxygen and food in what is known as a biological life support system. Additionally the waste from these processes could be used to make more carbon dioxide, recycling the nutrients, water and carbon. The Micro Ecological Life Support System Alternative (MELiSSA), designed by European Space Agency, is a model system of an advance biological life support system that would be based on different species of microbes and plants. Consisting of many bioreactors and plant chambers, MELiSSA would break down the organic waste from the crew and non-edible parts of the higher plants [22]. We can learn a lot about how MELiSSA works by looking at soil systems and processes here on Earth.
The MELiSSA loop is the basis of the life support system created by the ESA and uses processes we see all the time on earth. For example, the transformations of nitrogen (mineralization, nitrification, immobilization and denitrification) show the different ways nitrogen is transformed in soil and in the atmosphere. From studying how microbes interact in soil has helped us create MELiSSA, which combines multiple systems to produce food, water and oxygen for space explorers.
Energy Production
One example of the use of microbes in in the production of energy and additional waste management is the use of a MFC, or microbial fuel cell. MFCs can be used to generate electricity and process waste, but they currently produce low amounts of energy and depend on microbes processing organic matter in an anaerobic anode chamber [23]. On earth MFCs can be made using the soil found all around us because soil contains a diverse population of microbes, along with the nutrients needed by those microbes to generate energy.
Microbial Mineral Resource Extraction
Gold Production, Detection, and Transformation by Microbes
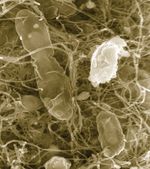
Some bacteria are capable of reducing toxic gold chloride into pure gold. Cupriavidus metallidurans is one of the few bacteria that can survive in high concentrations of heavy metal in mines throughout the world [24]. Although the bacteria only produce small amounts of gold nanoparticles too small to be profitable, they can be used by miners as biosensors to detect the concentrations of gold in environmental samples. Additionally, the gold nanoparticles synthesized by the bacteria could have potential in applications for many kinds of technology, such as optoelectronics, imaging technology, and drug delivery. Transcriptomic microarrays and DNA sequencing can detect bacterial genes that are associated with gold transformation, and thus pinpoint where significant gold deposits are present. There is great interest for the application of microbes in transforming toxic salts into gold, and scientists are studying both marine and terrestrial species to look for new insights into this area of geomicrobiology.
Role of Geomicrobiology in Bioleaching
Bioleaching can use microbes to extract metal from ore and remove metal impurities without the use of cyanide, mercury, or other chemicals. Biological forms of extraction can be cleaner than chemical methods because less toxins are released by the process, and detoxification can occur on site during processing. Chemolithotrophic bacteria reduce metal sulfides to metal sulfates, and heterotrophic bacteria or fungi exude organic acids, chelating, and complexing compounds to extract other ores and minerals. Although chemical extraction is still used when it is cheaper or another process is unknown, the field of bioleaching has high potential for further development.
The most active bacteria used in bioleaching are from genus Thiobacillus, gram negative bacteria that perform aerobic oxidation of sulfur compounds in acidic conditions. Other bioleaching genus’ include iron- oxidizing Leptospirillum and Thermophilic bacteria [25].
Role of Geomicrobiology in Removal and Disposal of Heavy Metals and Pollutants
Geomicrobiology can be applied to soil bioremediation, watershed reclamation, and to remove plumes of industrial contamination or radiation from the environment. Microbes in the soil and watertable are able to degrade contaminants that have no other practical source of removal and may be toxic to plants and other organisms. Inoculation of multiple target microbes can be used to remove multi-chemical pollutants from a site simultaneously[26]. Selection and application of species must take into consideration the reactions that will take place at each step, to ensure the target compound is cleaned from the soil; for example, the desired reactions may require anaerobic or aerobic conditions in the soil and thus the introduced microbes must survive and thrive in such conditions.
Extraction processes bring heavy metals and other deep subsurface compounds to the surface soil or to the watershed resulting in contamination. Processing metal ore and other resources for production with heavy metals, like mercury, also contribute to the pollution. Microbes found within these layers have evolved to breakdown, reduce, or remove these compounds in their natural settings to extract energy and nutrients through use of precipitation, redox, sorption, and metabolic processes. Target microbes are inoculated at contaminated sites and/or the conditions are changed to select for the desired native microbial community that will transform the pollutant[27]. Heavy metals including arsenic, zinc and sulfur can be precipitated out of acid mine wastewaters by sulfate-reducing bacteria, producing highly insoluble metal sulfides that are retained by wetland sediments[26].
Bacteria can reduce heavy metals from the soil in the rhizosphere for plant uptake and removal[28]. Toxins can be converted to nontoxic forms and remain in the soil or be removed by translocation from the roots up into the plant, which can then be harvested to remove the metal from the site. For example, by providing anoxic conditions, high concentrations of toxic chromium (VI) are reduced by rhizospheric bacteria Arthrobacter and Pseudomonas to form immobile, nontoxic Cr(III)[29]. Chromium can be reduced from toxic Cr(VI) to nontoxic Cr(III) by endophytic bacteria within their cells; these Cr compounds are absorbed into the plant for easy removal from the soil and groundwater through harvesting. Some examples of endophytic bacteria species capable of Cr reduction include Ralstonia pickettii and Ochrobactrum intermedium (both gram -), and other non-endophytic examples of Cr-reducing bacteria include Bacillus cereus and Streptomyces (both gram +)[28].
Role of Geomicrobiology in Radiation Contaminated Soils and Groundwater
Radiotrophic fungi can survive and colonize water and substrates (i.e. concrete barriers) in sites contaminated by severe radioactivity by using melanin pigment to transfer electrons and gain energy from the radioactive material. More study is needed to confirm if the radioactive material is then transformed to lower energy, safer products as the extra electrons are transferred by reduction. The fungal hyphae also deteriorate the concrete barriers by colonization and mineral weathering processes, which could result in release of radioactive material. A famous case is Chernobyl: after ten years of the catastrophe of Chernobyl in 1986 extensive fungal growth was observed on the walls and other building structures constructed in the inner part of the “Shelter” built over the fourth Unit of the Chernobyl nuclear power plant. Safe long-term storage of both existing and future nuclear waste is of vital importance in protecting the environment, therefore the study of these fungi has been key for future construction of nuclear waste repositories. Some of the microorganisms isolated from these extreme environments include modified strains from the genera Alternaria, Cladosporium, and Aureobasidium[30].
Role of Geomicrobiology in Agriculture
As agriculture enters the 21st century, new innovations are being discovered using microbes to increase production, yield, and sustainability in cropping systems. Soil quality and conservation are also becoming priorities on the agricultural forefront to combat the unintended consequences of conventional cropping systems. Metal and sulfate-reducing bacteria are responsible for precipitation and immobilization of metals like iron or manganese, and sulfate in the soil, determining the availability of these plant limiting nutrients[27].
For example, Phosphate solubilizing microorganisms (PSMs) use organic acids to dissociate phosphorus in small amounts from metal-phosphate compounds, making it available for plant uptake. PSM activity can result in reduced need for P fertilizer use and is being researched for use in biofertilizers. Some examples of PSMs include Chromobacterium, Azotobacter, and Bradyrhizobium[27]. Rhizobia have been used for some time to help increase Nitrogen fixation in soils, however increasing knowledge of microbial control of nutrient availability are broadening sustainable approaches to improved crop and soil health and quality.
Microorganisms can be used in integrated pest management systems to fend off pests. The bacterium Thiobacillus, a genus of 'Hydrogenophilaceae', are thermophilic Proteobacteria growing in temperatures up to 50 °C. They obtain their energy from hydrogen oxidation and are used as pest control in potato fields to control scabs[11]. Microbes are also used in less toxic insecticides called Microbials.
Current Research
Magnetotactic Bacteria
Magnetotactic bacteria produce small magnetite crystals to form microbial compasses. These materials are used in building microchips and electronics.
For example, a number of magnetic nanomaterials are being synthesized with biomimetic techniques, which were inspired by the mineralization processes of iron these magnetotactic bacteria did, and are used in, to name a few, "high-density data storage and ferrofluidic devices, electromagnetic shielding, spintronics, and quantum computing, and applications in diagnostic medicine and targeted drug delivery"[31].
Advances in Removal of Heavy Metals and Radioactivity
Research of microbial activities using radioactive and toxic metal waste in bedrock groundwater for safe long-term disposal is showing great potential for nuclear waste management. Corrosion of steel, copper, and other compounds is induced deep within the bedrock at greater rates than abiotic processes. The groundwater microbes bind the metal surfaces, forming biofilms resulting in corrosion of the metals[32].
References
- ↑ U.S. Geological Survey http://microbiology.usgs.gov/geomicrobiology.html
- ↑ 2.0 2.1 Gadd, G. M. 2010. Metals, minerals and microbes: geomicrobiology and bioremediation. Microbiology, 156(3), 609-643.
- ↑ Hall , J.C., Wickenden ,J.S., Kerrm Y. F. Yau. 2009. Biochemical Conjugation of Pesticides in Plants and Microorganisms: An Overview of Similarities and Divergences. Pesticide Biotransformation in Plants and Microorganisms. 5, 89-118.
- ↑ Herrera, A., Cockell, C. S., Self, S., Blaxter, M., Reitner, J., Arp, G., Drose, W., Thorsteinsson, T. , Tindle, A. G. 2008. Bacterial Colonization and Weathering of Terrestrial Obsidian in Iceland. Geomicro. J. 25: 25-37.
- ↑ Delgado, G. Delgado, R., Parraga, J., Rivadeneyra, M. A., Aranda, V. 2008. Precipitation of Carbonates and Phosphates by Bacteria in Extract Solutions from a Semi-arid Saline Soil. Influence of Ca2+ and Mg2+ Concentrations and Mg2+/Ca2+ Molar Ratio in Biomineralization. Geomicro. J. 25:1–13.
- ↑ Das, A. P., Sukla, L. B., Pradhan, N., & Nayak, S. 2011. Manganese biomining: a review.Bioresource technology, 102(16), 7381-7387.
- ↑ Murray, K.J., Tebo, B.M. 2007. Cr(III) Is Indirectly Oxidized by the Mn(II)-Oxidizing Bacterium Bacillus sp. Strain SG-1. Environ. Sci. Technol., 41, 528-533.
- ↑ Senko, J. M., Suflita, J. M., Krumholz, L. R. 2005. Geochemical Controls on Microbial Nitrate-Dependent U(IV) Oxidation. Geomicro. J. 22:371-378.
- ↑ The Environmental Literacy Council. Phosphorus cycle. http://enviroliteracy.org/air-climate-weather/biogeochemical-cycles/phosphorus-cycle/
- ↑ 10.0 10.1 Sylvia, D. M., Hartel, P. G., Fuhrmann, J. J., Zuberer, D. A. Principles of Applications of Soil Microbiology. 2nd edition. 2005. Pearson Prentice Hall.
- ↑ 11.0 11.1 Rosling, A., Suttle, K B., Johansson, E., Van Hees, P. A. W., Banfield, J. F. 2007. Phosphorous availability influences the dissolution of apatite by soil fungi. Geobiology 5 (3): 265–280.
- ↑ Klotz, M. G. (2011). BryantDA and Hanson TE (2011) The microbial sulfurcycle. The microbial sulfur cycle, 5.
- ↑ Campbell, B. J., Engel, A. S., Porter, M. L., & Takai, K. (2006). The versatile ε-proteobacteria: key players in sulphidic habitats. Nature Reviews Microbiology, 4(6), 458-468.
- ↑ Emerson, D., Roden, E., Twining, B.S. 2012. The microbial ferrous wheel: iron cycling in terrestrial, freshwater, and marine environments. Frontiers in Microbiolog
- ↑ 15.0 15.1 15.2 Colombo, C., Palumbo, G., He, J. Z., Pinton, R., & Cesco, S. (2014). Review on iron availability in soil: interaction of Fe minerals, plants, and microbes.Journal of soils and sediments, 14(3), 538-548.
- ↑ Lovley, D. R., Holmes, D. E., & Nevin, K. P. (2004). Dissimilatory fe (iii) and mn (iv) reduction.Advances in microbial physiology, 49, 219-286.
- ↑ Saha, R., Saha, N., Donofrio, R. S., & Bestervelt, L. L. (2013). Microbial siderophores: a mini review. Journal of basic microbiology, 53(4), 303-317.
- ↑ Kraemer, S. M. (2004). Iron oxide dissolution and solubility in the presence of siderophores. Aquatic sciences, 66(1), 3-18.
- ↑ 19.0 19.1 Sindhu, S. S., Phour, M., Choudhary, S. R., & Chaudhary, D. (2014). Phosphorus Cycling: prospects of using rhizosphere microorganisms for improving phosphorus nutrition of plants. In Geomicrobiology and biogeochemistry (pp. 199-237). Springer Berlin Heidelberg.
- ↑ Khan, M. S., Zaidi, A., & Ahmad, E. (2014). Mechanism of phosphate solubilization and physiological functions of phosphate-solubilizing microorganisms. In Phosphate Solubilizing Microorganisms (pp. 31-62). Springer International Publishing.
- ↑ Bonch-Osmolovskaya, E. A., Miroshnichenko, M. L., Slobodkin, A. I., Sokolova, T. G., Karpov, G. A., Kostrikina, N. A., ... & Pimenov, N. V. (1999). Biodiversity of anaerobic lithotrophic prokaryotes in terrestrial hot springs of Kamchatka. MICROBIOLOGY-AIBS-C/C OF MIKROBIOLOGIIA, 68, 343-351.
- ↑ Godia, F., Albiol, J., Montesinos, J. L., Pérez, J., Creus, N., Cabello, F., ... & Lasseur, C. (2002). MELISSA: a loop of interconnected bioreactors to develop life support in space. Journal of biotechnology, 99(3), 319-330.
- ↑ Cockell, C. S. (2010). Geomicrobiology beyond Earth: microbe–mineral interactions in space exploration and settlement. Trends in microbiology, 18(7), 308-314.
- ↑ Gwynne, P. (2013). Microbiology: There's gold in them there bugs. Nature, 495(7440), S12-S13.
- ↑ Bosecker, Klaus (1997). Bioleaching: metal solubilization by microorganisms. FEMS Microbiology Review 20 (pp. 591-604)
- ↑ 26.0 26.1 Sobolewski, A. 2006. A Review of Processes Responsible for Metal Removal in Wetlands Treating Contaminated Mine Drainage, International Journal of Phytoremediation, 1:1, 19-51.
- ↑ 27.0 27.1 27.2 N. Parmar and A. Singh (eds.), Geomicrobiology and Biogeochemistry, Soil Biology 39, DOI 10.1007/978-3-642-41837-2_11. Springer-Verlag Berlin Heidelberg 2014
- ↑ 28.0 28.1 Dimitroula, H., Syranidou, E., Manousaki, E., Nikolaidis, N. P., Karatzas, G. P., & Kalogerakis, N. (2015). Mitigation measures for chromium-VI contaminated groundwater–The role of endophytic bacteria in rhizofiltration. Journal of hazardous materials, 281, 114-120.
- ↑ Gutiérrez, A. M., Cabriales, J. J. P., & Vega, M. M. (2010). Isolation and characterization of hexavalent chromium-reducing rhizospheric bacteria from a wetland. International journal of phytoremediation, 12(4), 317-334.
- ↑ Fomina, M., Podgorsky, V. S., Olishevska, V., Kadoshnikov, V. M., Pisanska, I. R., Hillier, S., Gadd, G. M. 2007. Fungal Deterioration of Barrier Concrete used in Nuclear Waste Disposal. Geomicro. J. 24:643-653.
- ↑ Prozorov, T., Bazylinski, D. A., Mallapragada, S. K., & Prozorov, R. (2013). Novel magnetic nanomaterials inspired by magnetotactic bacteria: Topical review. Materials Science and Engineering: R: Reports, 74(5), 133-172.
- ↑ L. Carpén, P. Rajala, M. Bomberg, "Microbially Induced Corrosion in Deep Bedrock", Advanced Materials Research, Vol. 1130, pp. 75-78, Nov. 2015
Edited by students of Kate Scow