Engineering Salmonella for Cancer Treatment: Difference between revisions
Line 62: | Line 62: | ||
===Synchronized Lysis Circuits=== | ===Synchronized Lysis Circuits=== | ||
[[Image:Din.png|thumb|300px|right|Schematic of the synchronized lysis circuit. The bacterial population reaches the quorum threshold at a critical AHL concentration which then drives transcription of the <i>ɸX174E</i> lysis gene.]] | [[Image:Din.png|thumb|300px|right|Schematic of the synchronized lysis circuit. The bacterial population reaches the quorum threshold at a critical AHL concentration which then drives transcription of the <i>ɸX174E</i> lysis gene.<ref name=Din></ref>]] | ||
One such treatment involved genetic lysis circuits.<ref name=Din>Din, M.O. et al. (2016) ‘Synchronized cycles of bacterial lysis for in vivo delivery’, Nature, 536(7614), pp. 81–85. Available at: https://doi.org/10.1038/nature18930. | One such treatment involved genetic lysis circuits.<ref name=Din>Din, M.O. et al. (2016) ‘Synchronized cycles of bacterial lysis for in vivo delivery’, Nature, 536(7614), pp. 81–85. Available at: https://doi.org/10.1038/nature18930. | ||
Line 74: | Line 74: | ||
<br> | <br> | ||
[[Image:Din2.png|thumb|250px|left|Average tumor weight over time in mice injected with various cancer therapies. SLC-3, the triple therapy <i>S. typhimurium</i> strain, was most effective.]] | [[Image:Din2.png|thumb|250px|left|Average tumor weight over time in mice injected with various cancer therapies. SLC-3, the triple therapy <i>S. typhimurium</i> strain, was most effective.<ref name=Din></ref>]] | ||
While FNR-responsive promoters are induced by hypoxic conditions, there remain low levels of transcription in oxygenic conditions.<ref name=Khoroshilova>Khoroshilova, N., Beinert, H. and Kiley, P.J. (1995) ‘Association of a polynuclear iron-sulfur center with a mutant FNR protein enhances DNA binding.’, Proceedings of the National Academy of Sciences, 92(7), pp. 2499–2503. Available at: https://doi.org/10.1073/pnas.92.7.2499. | While FNR-responsive promoters are induced by hypoxic conditions, there remain low levels of transcription in oxygenic conditions.<ref name=Khoroshilova>Khoroshilova, N., Beinert, H. and Kiley, P.J. (1995) ‘Association of a polynuclear iron-sulfur center with a mutant FNR protein enhances DNA binding.’, Proceedings of the National Academy of Sciences, 92(7), pp. 2499–2503. Available at: https://doi.org/10.1073/pnas.92.7.2499. |
Revision as of 13:48, 15 April 2024
by Isaac Johnson '24
Introduction
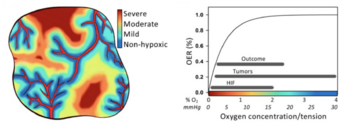
Cancer is perhaps the most notorious disease on Earth. Over decades of research, four major therapies have been developed: surgery, radiotherapy, chemotherapy, and immunotherapy.[1] However, cancerous cells that rapidly proliferate often outgrow the surrounding vasculature, developing regions of hypoxia.[2] Hypoxic conditions directly lead to treatment resistance of all therapies but surgery. Radiotherapy relies on the presence of molecular oxygen to generate reactive oxygen species to induce cell damage,[3] while chemo- and immunotherapeutic drugs are administered through the circulatory system, and therefore have inherently lower drug uptake with limited vasculature.[4] Furthermore, hypoxia reduces the cytotoxicity of these drugs and can trigger metastasis.
A solution may lie in bacterial-based treatments. Far from being a new discovery, bacterial treatments were observed by Dr. William Coley in the 19th century when injections of Streptococcus pyogenes led to tumor regression.[5] While the results reported by Coley were promising, many highlighted inconsistencies in his methods and doubted their legitimacy. Later, with the advent of radiotherapy in the 1890s and chemotherapy in the 1930s, bacterial treatments were forgotten. In hindsight, Coley’s experiments were an early, rudimentary form of immunotherapy. Tumors evade or suppress a patient’s immune system through various molecular pathways,[6] but an infection can naturally stimulate the immune system and trigger the body’s defense mechanisms against a tumor.
Unsurprisingly, bacteria alone are insufficient cancer treatments, but they have several advantages that are not found in current methods. Unlike chemotherapy and immunotherapy, many bacteria do not require the circulatory system to propagate within a host. Additionally, many bacteria are facultative anaerobes and thrive in hypoxic conditions. Not only would certain species be able to penetrate a tumor, but they could grow within the tumor microenvironment as well.[7] Unfortunately, bacteria’s innate stimulation of the immune system is often inefficient in eliminating cancer, unpredictable from patient to patient, and can cause several negative effects which may outweigh the possible benefits. However, genetic engineering is enabling researchers to inhibit a bacterium’s virulence, increase its localization to tumors, and implement novel treatment strategies based on chemo- and immunotherapeutic approaches.[8]
This page focuses on recent engineering efforts on Salmonella typhimurium, a gram-negative, rod-shaped pathogen. S. typhimurium is one of the leading causes of foodborne illness, known to cause gastroenteritis in humans and animals.[9] However, several characteristics make it a promising candidate for cancer therapy. It has flagella allowing for movement and is facultatively anaerobic, allowing it to thrive in hypoxic conditions. Additionally, it is an intracellular pathogen capable of infiltrating and reproducing inside host cells.[10] Lastly, and most importantly, it is a well-studied species with a fully sequenced genome.[11]
Safety and Specificity
Salmonella typhimurium is naturally retained in tumor microenvironments (TMEs) due to selective colonization: after initial dosing, the bacteria delivered to the tumor is approximately equal to the amount of bacteria colonizing surrounding tissues. However, outside of the immunosuppressive environment of the TME, the surrounding bacteria die while bacteria in the TME proliferate.[7] This results in an approximate 1,000-fold increase of tumor colonization compared to other tissues.[12] While this is clearly beneficial, S. typhimurium will also accumulate in healthy organs. For this reason, genetically-engineered strategies have been developed to increase specificity and safety.
Virulence Knockouts
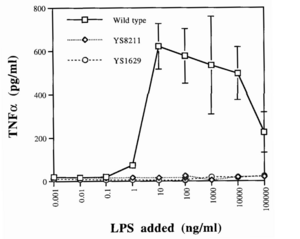
One such strategy involves knocking out certain genes of S. typhimurium. Gram-negative bacteria like Salmonella have a lipopolysaccharide (LPS) outer membrane—polysaccharide chains extending from the cell—that protects the cell from exterior toxins and bile salts.[14] These polysaccharides are rooted in the membrane by lipid A, a toxic molecule responsible for much of S. typhimurium’s pathogenicity. Upon bacterial lysis by the host’s immune system, lipid A is released from the membrane and triggers the expression of inflammatory proteins like TNFɑ.[15] While this pathway is essential for stimulating the immune system and eliminating infections, it is dangerous in cases of severe infection. A surplus of lipid A will prompt an overproduction of inflammatory factors, triggering blood clotting, septic shock, and possibly death.[16]
Since the LPS membrane is critical for bacterial survival, a given knockout strain must disarm the toxic capabilities of lipid A while retaining its role in membrane structure. By measuring TNFɑ induction between strains, researchers found that the deletion of the msbB gene in S. typhimurium produced viable cells with a 10-fold decrease in pathogenicity, along with retaining all of its previous tumor-targeting abilities.[13] Loss of the msbB gene prevents the myristoylation of lipid A, a lipid modification reaction crucial for downstream pathways.
This attenuated strain of S. typhimurium, named VNP20009, was isolated and tested in a mouse melanoma model, causing tumor regression.[13] It was subsequently implemented in clinical trials. These trials revealed that while VNP20009 was safe for patients, tumors were weakly colonized and no significant regressive effects were observed.[17] These results suggested that TNFɑ induction may have been critical to Salmonella’s antitumor properties, emphasizing the difficulty of achieving both safe and efficacious treatments.[8]
Auxotrophic Knockouts
Another strategy is to design auxotrophic strains of attenuated S. typhimurium: knockout strains unable to synthesize certain biomolecules necessary for growth, rendering them dependent on exterior sources. In addition to the msbB gene, VNP20009 also contains a purI gene knockout, rendering them dependent on exterior adenine.[13] Another strain of Salmonella, A1-R, was rendered auxotrophic for both leucine and arginine.[18][19] These molecules are enriched in the neoplastic tissue of tumors but not in regular tissue, thereby inhibiting growth outside the TME. Further testing in murine models showed significant tumor localization and regression of metastatic prostate cancer,[7] but safety concerns prevented A1-R from moving on to clinical trials.
Surface Proteins
Rather than knocking out certain genes, other studies seek to express exogenous surface protein moieties that enable specific binding to tumors. One such study expressed a camelid single-domain antibody (conjugated against human CD20 tumor antigens) on the surface of attenuated S. typhimurium.[20] Rather than a conventional antibody with a heavy and light chain, single-domain antibodies are naturally produced by camelids and are composed of a singular heavy chain.[21] These antibodies provide several advantages: they are more soluble in water,[22] more temperature-resistant,[23] and simply less bulky, allowing for greater tissue permeability.[24] The researchers cloned this single-domain antibody into lentivectors, which then inserted the sequence into the S. typhimurium genome.[20] Tumor localization and infection was significantly increased for CD20-expressing tumors both in vitro and in vivo.
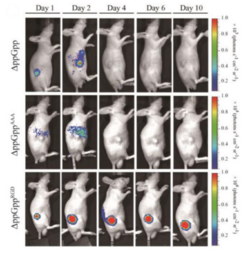
Another study modified a preexisting surface protein in Salmonella: the outer membrane protein A, or OmpA.[25] Researchers added a three residue sequence, R-G-D, to the outer loop of OmpA using a plasmid vector. The RGD peptide binds specifically to ɑvβ3 integrin, a receptor protein widely expressed on certain cancers during angiogenesis. Two other strains of S. typhimurium, one with wild-type OmpA and one with a triple alanine peptide in the outer loop, were engineered as controls to compare tumor-targeting abilities. The RGD peptide strain showed strong and prolonged localization in mouse models of human breast cancer.[25] Together, these results highlight the variety of strategies employed to increase the safety and specificity of S. typhimurium strains.
Treatment Mechanisms
While tumor localization is crucial for any cancer therapy, bacterial properties alone are not enough to cause significant tumor regression. Therefore, different treatment mechanisms against tumors are being engineered using Salmonella as a mode of delivery.
Synchronized Lysis Circuits
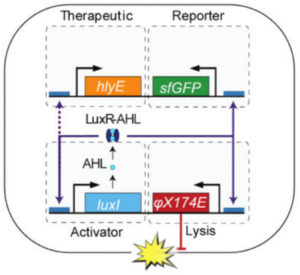
One such treatment involved genetic lysis circuits.[26] S. typhimurium is an intracellular pathogen, meaning it is capable of infiltrating and replicating within host cells. After localization to a tumor, genetically-programmed cell lysis can administer cytotoxic compounds directly inside the cancer cells. It is important to note that although Salmonella is contained in a vacuole inside the host cell, many toxins readily cross the membrane through pores and are able to elicit a biological effect. The researchers accomplished this programmed cell lysis by coupling previously characterized positive and negative feedback mechanisms[27] to generate quorum-sensing oscillatory dynamics, or a synchronized lysis circuit (SLC). A promoter, luxI, regulates the expression of an autoinducer, AHL. AHL binds with a LuxR cofactor, enabling luxI transcriptional induction and completing the positive feedback mechanism. AHL can translocate between neighboring cells, thereby synchronizing different circuits. The expression of the bacteriophage lysis gene ɸX174E is also regulated by the luxI promoter, leading to the negative feedback loop of cell lysis.
This SLC, carried on a plasmid, was transformed into attenuated S. typhimurium and tested in microfluidic devices to gauge population dynamics. As expected, researchers observed a slow buildup of bacteria until a quorum threshold was reached, triggering widespread lysis and releasing bacterial contents into the device. A small but consistent number of bacteria remained after each lysis event to proliferate and start the cycle again. Naturally, the next step was to heterologously express cytotoxic compounds in the SLC strain of S. typhimurium. The cytotoxic compound they selected was hemolysin E, or HlyE.
An earlier study designed a novel, hypoxia-induced promoter fused to the cytotoxic protein HlyE.[28] As a facultative anaerobe, S. typhimurium naturally expresses FNR, a transcription factor. This protein dimerizes in the presence of iron-sulfur ion clusters which only form in the absence of oxygen.[29] The formation of these clusters—and the dimerized complex that results—increases binding to DNA and recruitment of RNA polymerase. Therefore, FNR-responsive promoters are induced by hypoxic conditions, making these promoters ideal regulators of hlyE expression.
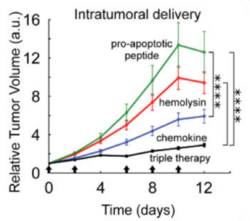
While FNR-responsive promoters are induced by hypoxic conditions, there remain low levels of transcription in oxygenic conditions.[30] Before fusing the promoter to a cytotoxic compound, researchers sought to mutate the promoter so transcription is exclusively activated in the hypoxic conditions of a tumor. FF+20, an FNR-responsive promoter in E. coli, was chosen for the study. The -10 promoter element was mutated, lowering transcription levels but increasing dependence on FNR. The novel FNR-dependent promoter, FF+20*, was then fused to hlyE, encoding for the cytotoxic protein hemolysin E.[28] Hemolysin E, derived from E. coli, is a pore-forming toxin shown to have anti-tumor effects.[31]
This FF+20*-hlyE construct was cloned into plasmids and transformed into the SLC strain of S. typhimurium, giving the new strain (SLC-hly) the cytotoxic capability crucial for tumor regression.[26] When HeLa cancer cells were cocultured with SLC-hly, they observed cell death after bacterial lysis, showing efficient toxin production and release. However, in vivo experiments with mice showed little tumor regression despite oscillatory bacterial lysis being observed. To combat this, they generated two other SLC strains containing different compounds: one with mCCL21 for host immune cell recruitment,[32] and one with CDD-iRGD to trigger tumor cell apoptosis.[33] Interestingly, the latter construct uses the pro-apoptotic protein (CDD) fused to the same RGD peptide discussed in Section 2.3 that binds to the ɑvβ3 integrin receptor on tumors.[25] An equal mixture of all three strains, dubbed SLC-3, elicited the strongest in vivo tumor regression than any singular strain. Unfortunately, it also proved to be more lethal than its SLC-hly counterpart, highlighting the difficult balance between potency and safety in cancer treatments.
Type III Secretion Systems
Small molecules, like the hemolysin E toxin discussed in Section 3.1, typically lack specificity. This is, in part, what contributes to the difficult balance between potency and safety in cancer treatments. Highly specific binding molecules such as antibodies are ideal, yet they cannot cross cell membranes and therefore cannot affect host cells.[34] Rather than programmed cell lysis, other researchers sought to utilize the type III secretion system (T3SS) innately expressed in Salmonella to bypass the cell membrane (and the host vacuolar membrane) and administer a highly specific binding molecule to tumors.[35]
T3SSs are found in many gram-negative bacteria, and likely evolved as a virulence mechanism.[36] The protein complex forms a needle-like structure which inserts into cell membranes, providing a path for certain proteins to enter the host cytoplasm. The entire apparatus is made of 20 to 25 different proteins, half of which are conserved across all T3SS species.[37] Various proteins form a ring in the inner and outer bacterial membranes, creating openings for the needle-like protrusion to form. The needle itself is approximately 80 nanometers long, with anywhere from 10 to 100 needles on each bacterium. The needle is composed of helical proteins MxiH, 9 kDa, and PrgI, 9 kDa.[38] These protein subunits polymerize to form a rigid, hollow pipeline with a 25 Å inner diameter.[39] Since fully folded proteins typically range from 20 to 30 Å, proteins are either partially folded or unfolded when passing through the apparatus.
In a 2006 study, researchers engineered an avirulent strain of S. typhimurium to secrete tumor-specific antigens through the T3SS.[35] Upon antigen binding, the host's immune antibodies would be recruited to the site and launch an immune response, attacking the tumor. The antigen targeted NY-ESO-1, a protein often expressed in tumors but absent in normal cells. The entire amino acid sequence of the antigen was cloned on a plasmid and inserted into S. typhimurium. They first confirmed that the bacteria successfully secreted the antigen in vitro. Then, a human melanoma cell line, SK-MEL-21, was infected with the bacteria. Specific binding was visualized with immunohistochemistry using NY-ESO-1 antibodies, yielding promising results: after oral administration of the S. typhimurium strain to mice with NY-ESO-expressing tumors, antigen-specific T cells were generated and led to a complete regression of the tumors.[35] The most obvious limitation of this study is its scope. It is only effective against tumors expressing the NY-ESO-1 antigen. However, after priming mice with NY-ESO-1 DNA, tumors that did not initially express NY-ESO-1 were eradicated after bacterial infection. Although this method has limited clinical applications with human patients, the straightforward nature of T3SS has inspired many other studies.
A more recent effort focused on engineering a T3SS in Salmonella that secretes DARPins rather than antigens.[40] DARPins, or Designed Ankyrin Repeat Proteins, are engineered, highly specific binding proteins which mitigate several weaknesses of traditional antibodies. Antibodies are expensive, difficult to make, and have low tissue penetration due to their approximate 150 kDa weight.[41] DARPins, however, are cheaper to make and typically range from 14 to 21 kDa, allowing for deeper tissue penetration. As their name suggests, they are derived from ankyrin repeats, an abundant structural motif used in many endogenous biological functions. Each motif is composed of two alpha helices connected by a variable chain. Each is made of 33 amino acids, seven of which form the highly variable chain. These small monomers form a protein scaffold which readily polymerizes, forming a repeat domain which varies in size depending on the length of the polymer.[42] The full DARPin is capped on both ends, and typically contains two to four library modules (units with a designed variable chain) in between. The alpha helical scaffold provides stability while the variable chains form a highly specific binding surface.
Therefore, researchers engineered a strain of S. typhimurium to deliver DARPins into the host cell’s cytoplasm using T3SS.[40] They utilized a pCASP plasmid system in which DARPin expression is regulated by the pSicA promoter, the same promoter which controls T3SS proteins. DARPin was conjugated against GFP to allow for visualization. Additionally, the DARPin protein itself was fused to Spt120, a fragment of a native secretion protein. This fragment acts as a tag so that DARPin is indeed recruited and secreted by the T3SS. HilA, an inducer of T3SS genes, was also included on the plasmid under an arabinose-inducible promoter (pBAD). Using this pCASP-HilA vector, they observed a significant increase in DARPin secretion into cultured HeLa human cervical cancer cells.
Despite their stability, DARPins unfold to pass through the narrow, 25 Å diameter needle and refold in the host cell’s cytoplasm.[43] Due to this, the researchers needed to confirm that DARPins still held full functionality after secretion. Therefore, GFP was fused to either Sec61, an endoplasmic reticulum protein, or H2B, a nuclear histone protein, in HeLa cells.[40] In both cases, the anti-GFP DARPins correctly localized to the endoplasmic reticulum and nucleus, confirming their functionality after refolding. Lastly, they incorporated previously-developed DARPins conjugated against the RAS signaling pathway[44] into the strain of S. typhimurium. The RAS signaling pathway is crucial for cell proliferation and growth. Since mutations in this pathway can often lead to cancer, it is an attractive therapeutic target.
One DARPin, dubbed K27, targets RAS-GTPase; the other, dubbed K55, targets the RAF cofactor. Upon binding, these DARPins were found to block the active site and inhibit RAS signaling. Downstream phosphorylation of several proteins—ERK1/2, AKT, and GSK3β—all decreased, indicative of reduced RAS activity.[40] While these are promising results, in vivo studies have yet to be conducted.
Conclusion
Hypoxic tumors are known to have resistance against three of the four modern cancer therapies.[1] Radiotherapy generates reactive oxygen species to induce cell damage; with oxygen absent, reactive oxygen species cannot form.[3] Chemo- and immunotherapeutic drugs are administered through the circulatory system, limiting drug uptake to vascularized regions of a tumor.[4] Surprisingly, bacteria like Salmonella typhimurium offer a possible solution.
S. typhimurium is a facultative anaerobe which readily permeates and proliferates in hypoxic tissue. Additionally, due to the immunosuppressive conditions of the TME, bacteria colonizing healthy tissues are cleared while bacteria colonizing the tumor continue to proliferate.[45] Eventually, the bacteria may elicit the host’s immune response, leading to tumor regression. Of course, these effects of Salmonella are insufficient, inconsistent, and therefore inappropriate for clinical use. However, since it is a highly studied species with a fully sequenced genome, there are current efforts to engineer different strains that increase its tumor localization and treatment mechanisms, cultivating the possiblity of bacterial-based therapeutics.
As it stands, several biological and logistic obstacles prevent bacterial-based cancer therapy from widespread use. While significant tumor regression has been seen in vitro and in mice, the same level of regression has not been achieved in humans (in the few clinical trials that have been conducted). As is true with other therapies, there is a balance between patient safety and anticancer potency that varies from case to case, and there is less control over dosages when utilizing bacterial modes of delivery. Finally, as is true with any new treatment, there are still gaps in the knowledge and a lack of data to inform future decisions. Luckily, research will continue to expand and give new insights, broadening our horizons of what is possible with cancer treatments.
References
- ↑ 1.0 1.1 1.2 Hompland, T., Fjeldbo, C.S. and Lyng, H. (2021) ‘Tumor Hypoxia as a Barrier in Cancer Therapy: Why Levels Matter’, Cancers, 13(3), p. 499. Available at: https://doi.org/10.3390/cancers13030499
- ↑ Chen, Z. et al. (2023) ‘Hypoxic microenvironment in cancer: molecular mechanisms and therapeutic interventions’, Signal Transduction and Targeted Therapy, 8(1), p. 70. Available at: https://doi.org/10.1038/s41392-023-01332-8
- ↑ 3.0 3.1 Menegakis, A. et al. (2021) ‘Resistance of Hypoxic Cells to Ionizing Radiation Is Mediated in Part via Hypoxia-Induced Quiescence’, Cells, 10(3), p. 610. Available at: https://doi.org/10.3390/cells10030610.
- ↑ 4.0 4.1 Ho, Y.-J., Thao, D.T. and Yeh, C.-K. (2022) ‘Overcoming Hypoxia-Induced Drug Resistance via Promotion of Drug Uptake and Reoxygenation by Acousto–Mechanical Oxygen Delivery’, Pharmaceutics, 14(5), p. 902. Available at: https://doi.org/10.3390/pharmaceutics14050902.
- ↑ McCarthy, E.F. (no date) ‘THE TOXINS OF WILLIAM B. COLEY AND THE TREATMENT OF BONE AND SOFT-TISSUE SARCOMAS’.
- ↑ Tie, Y. et al. (2022) ‘Immunosuppressive cells in cancer: mechanisms and potential therapeutic targets’, Journal of Hematology & Oncology, 15(1), p. 61. Available at: https://doi.org/10.1186/s13045-022-01282-8.
- ↑ 7.0 7.1 7.2 Zhou, S. et al. (2018) ‘Tumour-targeting bacteria engineered to fight cancer’, Nature Reviews Cancer, 18(12), pp. 727–743. Available at: https://doi.org/10.1038/s41568-018-0070-z.
- ↑ 8.0 8.1 Gurbatri, C.R., Arpaia, N. and Danino, T. (2022) ‘Engineering bacteria as interactive cancer therapies’, Science, 378(6622), pp. 858–864. Available at: https://doi.org/10.1126/science.add9667.
- ↑ Fàbrega, A. and Vila, J. (2013) ‘Salmonella enterica Serovar Typhimurium Skills To Succeed in the Host: Virulence and Regulation’, Clinical Microbiology Reviews, 26(2), pp. 308–341. Available at: https://doi.org/10.1128/CMR.00066-12.
- ↑ Guo, Y. et al. (2020) ‘Targeted cancer immunotherapy with genetically engineered oncolytic Salmonella typhimurium’, Cancer Letters, 469, pp. 102–110. Available at: https://doi.org/10.1016/j.canlet.2019.10.033.
- ↑ McClelland, M. et al. (2001) ‘Complete genome sequence of Salmonella enterica serovar Typhimurium LT2’, Nature, 413(6858), pp. 852–856. Available at: https://doi.org/10.1038/35101614.
- ↑ Sieow, B.F.-L. et al. (2021) ‘Tweak to Treat: Reprograming Bacteria for Cancer Treatment’, Trends in Cancer, 7(5), pp. 447–464. Available at: https://doi.org/10.1016/j.trecan.2020.11.004.
- ↑ 13.0 13.1 13.2 13.3 Low, K.B. et al. (1999) ‘Lipid A mutant Salmonella with suppressed virulence and TNFα induction retain tumor-targeting in vivo’, Nature Biotechnology, 17(1), pp. 37–41. Available at: https://doi.org/10.1038/5205.
- ↑ Bertani, B. and Ruiz, N. (2018) ‘Function and Biogenesis of Lipopolysaccharides’, EcoSal Plus. Edited by J.M. Slauch, 8(1), p. 10.1128/ecosalplus.ESP-0001–2018. Available at: https://doi.org/10.1128/ecosalplus.esp-0001-2018.
- ↑ Raetz, C.R.H. and Whitfield, C. (2002) ‘Lipopolysaccharide Endotoxins’, Annual Review of Biochemistry, 71(1), pp. 635–700. Available at: https://doi.org/10.1146/annurev.biochem.71.110601.135414.
- ↑ Esmon, C.T. (2000) ‘Regulation of blood coagulation’, Biochimica et Biophysica Acta (BBA) - Protein Structure and Molecular Enzymology, 1477(1–2), pp. 349–360. Available at: https://doi.org/10.1016/S0167-4838(99)00266-6.
- ↑ Toso, J.F. et al. (2007) ‘Phase I Study of the Intravenous Administration of Attenuated Salmonella typhimurium to Patients With Metastatic Melanoma’.
- ↑ Zhao, M. et al. (no date) ‘Tumor-targeting bacterial therapy with amino acid auxotrophs of GFP-expressing Salmonella typhimurium’, MEDICAL SCIENCES [Preprint].
- ↑ Zhao, M. et al. (2007) ‘Monotherapy with a tumor-targeting mutant of Salmonella typhimurium cures orthotopic metastatic mouse models of human prostate cancer’, Proceedings of the National Academy of Sciences, 104(24), pp. 10170–10174. Available at: https://doi.org/10.1073/pnas.0703867104.
- ↑ 20.0 20.1 Massa, P.E. et al. (2013) ‘Salmonella engineered to express CD20-targeting antibodies and a drug-converting enzyme can eradicate human lymphomas’, Blood, 122(5), pp. 705–714. Available at: https://doi.org/10.1182/blood-2012-12-474098.
- ↑ Harmsen, M.M. and De Haard, H.J. (2007) ‘Properties, production, and applications of camelid single-domain antibody fragments’, Applied Microbiology and Biotechnology, 77(1), pp. 13–22. Available at: https://doi.org/10.1007/s00253-007-1142-2.
- ↑ Stanfield, R.L. et al. (2004) ‘Crystal Structure of a Shark Single-Domain Antibody V Region in Complex with Lysozyme’, Science, 305(5691), pp. 1770–1773. Available at: https://doi.org/10.1126/science.1101148.
- ↑ Van Der Linden, R.H.J. et al. (1999) ‘Comparison of physical chemical properties of llama VHH antibody fragments and mouse monoclonal antibodies’, Biochimica et Biophysica Acta (BBA) - Protein Structure and Molecular Enzymology, 1431(1), pp. 37–46. Available at: https://doi.org/10.1016/S0167-4838(99)00030-8.
- ↑ Monegal, A. et al. (2009) ‘Immunological applications of single-domain llama recombinant antibodies isolated from a naïve library’, Protein Engineering, Design and Selection, 22(4), pp. 273–280. Available at: https://doi.org/10.1093/protein/gzp002.
- ↑ 25.0 25.1 25.2 25.3 Park, S.-H. et al. (2016) ‘RGD Peptide Cell-Surface Display Enhances the Targeting and Therapeutic Efficacy of Attenuated Salmonella -mediated Cancer Therapy’, Theranostics, 6(10), pp. 1672–1682. Available at: https://doi.org/10.7150/thno.16135.
- ↑ 26.0 26.1 26.2 26.3 Din, M.O. et al. (2016) ‘Synchronized cycles of bacterial lysis for in vivo delivery’, Nature, 536(7614), pp. 81–85. Available at: https://doi.org/10.1038/nature18930.
- ↑ Danino, T. et al. (2010) ‘A synchronized quorum of genetic clocks’, Nature, 463(7279), pp. 326–330. Available at: https://doi.org/10.1038/nature08753.
- ↑ 28.0 28.1 Ryan, R.M. et al. (2009) ‘Bacterial delivery of a novel cytolysin to hypoxic areas of solid tumors’, Gene Therapy, 16(3), pp. 329–339. Available at: https://doi.org/10.1038/gt.2008.188.
- ↑ Green, J. et al. (1996) ‘Reconstitution of the [4Fe-4S] cluster in FNR and demonstration of the aerobic-anaerobic transcription switch in vitro’, Biochemical Journal, 316(3), pp. 887–892. Available at: https://doi.org/10.1042/bj3160887.
- ↑ Khoroshilova, N., Beinert, H. and Kiley, P.J. (1995) ‘Association of a polynuclear iron-sulfur center with a mutant FNR protein enhances DNA binding.’, Proceedings of the National Academy of Sciences, 92(7), pp. 2499–2503. Available at: https://doi.org/10.1073/pnas.92.7.2499.
- ↑ Chiang, C.-J. and Huang, P.-H. (2021) ‘Metabolic engineering of probiotic Escherichia coli for cytolytic therapy of tumors’, Scientific Reports, 11(1), p. 5853. Available at: https://doi.org/10.1038/s41598-021-85372-6.
- ↑ Loeffler, M. et al. (2009) ‘Salmonella typhimurium engineered to produce CCL21 inhibit tumor growth’, Cancer Immunology, Immunotherapy, 58(5), pp. 769–775. Available at: https://doi.org/10.1007/s00262-008-0555-9.
- ↑ Chen, R. et al. (2013) ‘Application of a Proapoptotic Peptide to Intratumorally Spreading Cancer Therapy’, Cancer Research, 73(4), pp. 1352–1361. Available at: https://doi.org/10.1158/0008-5472.CAN-12-1979.
- ↑ Slastnikova, T.A. et al. (2018) ‘Targeted Intracellular Delivery of Antibodies: The State of the Art’, Frontiers in Pharmacology, 9, p. 1208. Available at: https://doi.org/10.3389/fphar.2018.01208.
- ↑ 35.0 35.1 35.2 Nishikawa, H. (2006) ‘In vivo antigen delivery by aSalmonella typhimurium type III secretion system for therapeutic cancer vaccines’, Journal of Clinical Investigation, 116(7), pp. 1946–1954. Available at: https://doi.org/10.1172/JCI28045.
- ↑ Coburn, B., Sekirov, I. and Finlay, B.B. (2007) ‘Type III Secretion Systems and Disease’, Clinical Microbiology Reviews, 20(4), pp. 535–549. Available at: https://doi.org/10.1128/CMR.00013-07.
- ↑ Ghosh, P. (2004) ‘Process of Protein Transport by the Type III Secretion System’, Microbiology and Molecular Biology Reviews, 68(4), pp. 771–795. Available at: https://doi.org/10.1128/MMBR.68.4.771-795.2004.
- ↑ Kubori, T. et al. (1998) ‘Supramolecular Structure of the Salmonella typhimurium Type III Protein Secretion System’, Science, 280(5363), pp. 602–605. Available at: https://doi.org/10.1126/science.280.5363.602.
- ↑ Loquet, A. et al. (2012) ‘Atomic model of the type III secretion system needle’, Nature, 486(7402), pp. 276–279. Available at: https://doi.org/10.1038/nature11079.
- ↑ 40.0 40.1 40.2 40.3 Chabloz, A. et al. (2020) ‘Salmonella-based platform for efficient delivery of functional binding proteins to the cytosol’, Communications Biology, 3(1), p. 342. Available at: https://doi.org/10.1038/s42003-020-1072-4.
- ↑ Stumpp, M.T., Binz, H.K. and Amstutz, P. (2008) ‘DARPins: A new generation of protein therapeutics’, Drug Discovery Today, 13(15–16), pp. 695–701. Available at: https://doi.org/10.1016/j.drudis.2008.04.013.
- ↑ Forrer, P. et al. (2003) ‘A novel strategy to design binding molecules harnessing the modular nature of repeat proteins’, FEBS Letters, 539(1–3), pp. 2–6. Available at: https://doi.org/10.1016/S0014-5793(03)00177-7.
- ↑ Wetzel, S.K. et al. (2008) ‘Folding and Unfolding Mechanism of Highly Stable Full-Consensus Ankyrin Repeat Proteins’, Journal of Molecular Biology, 376(1), pp. 241–257. Available at: https://doi.org/10.1016/j.jmb.2007.11.046.
- ↑ Guillard, S. et al. (2017) ‘Structural and functional characterization of a DARPin which inhibits Ras nucleotide exchange’, Nature Communications, 8(1), p. 16111. Available at: https://doi.org/10.1038/ncomms16111.
- ↑ Li, Z. et al. (2021) ‘Chemically and Biologically Engineered Bacteria‐Based Delivery Systems for Emerging Diagnosis and Advanced Therapy’, Advanced Materials, 33(38), p. 2102580. Available at: https://doi.org/10.1002/adma.202102580.
Authored for BIOL 238 Microbiology, taught by Joan Slonczewski,at Kenyon College,2024