Infection Mechanism of Genus Ebolavirus: Difference between revisions
Line 86: | Line 86: | ||
[[Image:Ebola Macrophage.gif|thumb|250px|right|<b>Figure 4</b>. Ebolavirus causes destabilization of the vascular endothelium leading to hemorrhage.]] | [[Image:Ebola Macrophage.gif|thumb|250px|right|<b>Figure 4</b>. Ebolavirus causes destabilization of the vascular endothelium leading to hemorrhage.]] | ||
Ebola virions then enter the endothelial cells through interactions between its surface GP and receptors in the lipid raft microdomains (Bavari et al. 2002). They move throughout the cell via microtubules or actin filaments until they begin to undergo transcription and translation (Yonezawa et al. 2005). Some of the products of this process are used to build new virions, while others, like sGP, are used to maximize the time Ebola can use a cell before its death (Simmons et al. 2002). During this process, Ebola GP is causing the cell to detach from those around it via interactions with VE-cadherin, as well as lose contact with its basement membrane thanks to interactions with integrin and CAMs (Wahl-Jensen et al. 2005). The newly created particles leave via lipid rafts. Due to this process, the vascular endothelium of the body is destabilized, which leads to the massive blood loss, which causes or relates to many of the symptoms, that is characteristic of Ebola patients (Bavari et al. 2002). | Ebola virions then enter the endothelial cells through interactions between its surface GP and receptors in the lipid raft microdomains (Bavari et al. 2002). They move throughout the cell via microtubules or actin filaments until they begin to undergo transcription and translation (Yonezawa et al. 2005). Some of the products of this process are used to build new virions, while others, like sGP, are used to maximize the time Ebola can use a cell before its death (Simmons et al. 2002). During this process, Ebola GP is causing the cell to detach from those around it via interactions with VE-cadherin, as well as lose contact with its basement membrane thanks to interactions with integrin and CAMs (Wahl-Jensen et al. 2005). The newly created particles leave via lipid rafts. Due to this process, the vascular endothelium of the body is destabilized, which leads to the massive blood loss, which causes or relates to many of the symptoms, that is characteristic of Ebola patients (Bavari et al. 2002, Fig. 4). | ||
During this process, the immune system is going haywire. Interferons are not being made (Basler et al. 2003). T cells cannot recognize that there is a problem (Sullivan et al. 2005). White blood cells cannot get to their necessary location because sGP prevents their movement (Wahl-Jensen et al. 2005). Macrophages and monocytes are releasing massive amounts of proinflammatory cytokines that are aiding in the destruction of the vascular endothelium, while also causing activation of the coagulation cascade. This cascade activates fibrinogenic and fibrinolytic pathways. Fibrin is a protein that is involved in the clotting of blood, so these pathways involve the building up or breaking down of fibrin meshes that form blood clots. These pathways eventually lead to disseminated intravascular coagulation, a state where the blood starts to coagulate around the body and use up platelets and coagulating factors. This puts the body in a paradoxical state where the organism can either die of hypovolemic shock from massive hemorrhage, or from catastrophic thrombosis, the formation of blood clots around the body (Geisbert et al. 2003). | During this process, the immune system is going haywire. Interferons are not being made (Basler et al. 2003). T cells cannot recognize that there is a problem (Sullivan et al. 2005). White blood cells cannot get to their necessary location because sGP prevents their movement (Wahl-Jensen et al. 2005). Macrophages and monocytes are releasing massive amounts of proinflammatory cytokines that are aiding in the destruction of the vascular endothelium, while also causing activation of the coagulation cascade. This cascade activates fibrinogenic and fibrinolytic pathways. Fibrin is a protein that is involved in the clotting of blood, so these pathways involve the building up or breaking down of fibrin meshes that form blood clots. These pathways eventually lead to disseminated intravascular coagulation, a state where the blood starts to coagulate around the body and use up platelets and coagulating factors. This puts the body in a paradoxical state where the organism can either die of hypovolemic shock from massive hemorrhage, or from catastrophic thrombosis, the formation of blood clots around the body (Geisbert et al. 2003). |
Revision as of 03:00, 3 November 2010
A Viral Biorealm page on the family Infection Mechanism of Genus Ebolavirus
Ebola hemorrhagic fever is a severe, often fatal, disease in humans and non-human primates caused by Ebolavirus (Fig. 1). Even though the virus first appeared in the Democratic Republic of the Congo (formerly Zaire) in the summer of 1976 and has only sporadically reemerged, it has still managed to capture the attention of the world due to death rates that can be as high as 90% as well as the visceral manner in which it kills (CDC 2010, Johnson & Breman 1978). Few viruses have the ability to turn internal organs into a soup that promptly flows out of the body, so those that do tend to capture the public eye.
Despite the low incidence of infections, the lethality and potential airborne transmission of Ebolavirus makes it a severe biological threat. This is compounded by the possibility of the virus being obtained from the wild for use as a biological weapon (Borio et al. 2002). As of present, there are no licensed vaccines or specific antiviral treatments available for Ebolavirus infections, with significant progress only made in the development of vaccines for nonhuman primates (Pratt et al. 2010). Therefore, the elucidation of the viral replication cycle as well as complete understanding of viral proteins is necessary for the production of human vaccines.
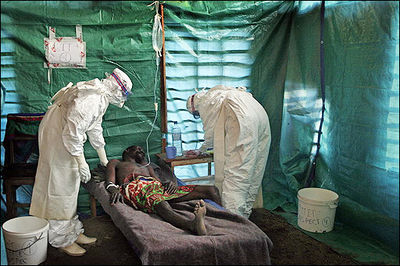
Ebola Hemorrhagic Fever
One of the reasons that Ebola is so dangerous is that its symptoms are varied and appear quickly, yet are close enough to those of other viruses that Ebola is not rapidly recognized. Initial symptoms include high fever, severe headache, severe weakness, exhaustion, sore throat, nausea, dizziness and muscle, joint or abdominal pain. The unfortunate thing about these symptoms is that they are easily mistaken for malaria, typhoid fever, dysentery, influenza or various bacterial infections, all of which are far more common in regions where Ebola is prevent, but also less fatal (CDC 2010).
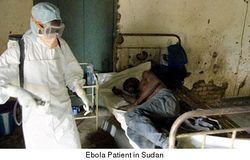
After this period, the fever often progresses to cause more serious symptoms: diarrhea, dark or bloody feces, vomiting blood, red eyes due to distention and hemorrhage of sclerotic arterioles, petechia, maculopapular rash and purpura. There is often gastrointestinal bleeding from the mouth and rectum, sometimes leading to the sloughing of the gut and venting from the anus. Internal and external hemorrhage from orifices, such as the nose and mouth, may also occur (Fig. 2). As the virus progresses, bleeding in the brain can lead to severe depression, seizures and delirium (CDC 2010).
The span of time from onset of symptoms to death is usually between 7 and 14 days. By the second week of the infection, the patient will either experience a full recovery or undergo systemic multi-organ failure. Mortality rates are generally high, ranging from 50-90% depending on the specific strain. The cause of death is normally due to hypovolemic shock or organ failure (WHO 2010).
Virion Structure
Filoviridae, of which Ebolavirus is a member, is a family of viruses that contain single, linear, negative-sense ssRNA genomes. The family name was derived from the Latin word filum, which alludes to the thread-like appearance of the virions when viewed under an electron microscope. Filoviruses have been divided into two genera: Ebola-like viruses with species Zaire, Sudan, Reston, Cote d’Ivoire and Bundibugyo; and Marburg-like viruses with the single species Marburg. All of these are responsible for hemorrhagic fevers in primates that are characterized by often fatal bleeding and coagulation abnormalities (Klenk & Feldmann 2004).
The tubular Ebola virions are generally 80 nm in diameter and 800 nm long. In the center of the particle is the viral nucleocapsid which consists of the helical ssRNA genome wrapped about the NP, VP35, VP30 and L proteins. This structure is then surrounded by an outer viral envelope derived from the host cell membrane that is studded with 10 nm long viral glycoprotein (GP) spikes. Between the capsid and envelope are viral proteins VP40 and VP24 (Feldmann et al. 1993).
The genome of each virion is around 19kb in length, and codes for seven structural and one non-structural protein. The gene order is as follows: 3′ – leader – NP – VP35 – VP40 – GP/sGP – VP30 – VP24 – L – trailer – 5′ (Fig. 3). The leader and trailer regions are not transcribed, but carry important signals that control transcription, replication and packaging of the genome into new virions (Crary et al. 2003). The seven genes each consist of their respective open reading frame as well as long non-translated sequences of unknown purpose that flank the coding regions (Klenk & Feldmann 2004).
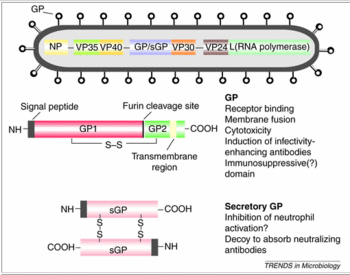
The envelope GP spike is the only viral surface protein, and thus has been implicated in cellular entry by mediating receptor binding and membrane fusion. It has also been shown as the crucial factor for Ebolavirus pathogenicity (Yonezawa et al. 2005). The product of the third gene, VP40, is located beneath the viral envelope where it helps to maintain structural integrity. It has also been associated with late endosomes and likely mediates filovirus budding due to its ability to induce its own release from cells in the absence of all other viral proteins (Jasenosky et al. 2001).
The second matrix protein, VP24, has been shown to suppress interferon production, and may also be important in regulation prior to budding. However, interferon interference is not the only function of VP24. Other experiments have shown that this protein, along with VP35 and NP are sufficient to form nucleocapsid structures (Huang et al. 2002). Lastly, VP24 is necessary for the correct assembly of a functional nucleocapsid, as lack of VP24 leads to reduced transcription and/or translation of VP30 (Hoenen et al. 2006).
The remaining structural proteins form the nucleocapsid, and are thus intimately associated with the viral genome. These are the nucleoprotein NP, the polymerase cofactor VP35, the viral-specific transcription activator VP30 and the viral RNA polymerase L. These nucleocapsid proteins have a dual function in the viral replication cycle: they are involved as structural components, but also catalyze replication and transcription of the genome. While NP, VP35 and L are sufficient for replication, transcription initiation will not proceed without VP30 (Mühlberger et al. 1999).
Ebolavirus genomes code for an additional protein, the non-structural secreted GP (sGP). This truncated protein is also encoded by the fourth gene, but synthesis is due to translation from non-edited mRNA species. The structural form requires a reading frame shift or transcriptional editing of a single nucleotide. Therefore, almost 80% of the proteins encoded by this gene are in the sGP form (Simmons et al. 2002). This protein is not found in virus particles, but is instead secreted from infected cells into the blood (Volchkov et al. 1995).
NEED TO TALK MORE ABOUT GP AND sGP
Immune System Evasion
One of the reasons that Ebola is so deadly is that it has multiple ways of interfering with or avoiding the human immune system. While the virus is busy destroying the human body, the immune system is either still in the process of discovering that there is a problem, or is in such disarray that it would be next to impossible to mobilize a unified effort to fight off the invader. This virus is able to hijack antibodies, take control of macrophages, inhibit movement of neutrophils, block interferon and destroy dendritic cells.
Antibodies normally function by attaching to their respective antigen, then starting a complement cascade that ultimately leads to the pathogen’s destruction by phagocytosis. After two IgG antibodies bind the GP spikes, complement component one (C1) binds the complex via the Fc portion of the antibodies. This complex then attaches to a C1q ligand on the surface of cells. While this normally promotes endocytosis of the target antigen by intracellular signaling, in this case it only serves in the binding of Ebola to viral receptors. C1q ligands have been identified on many cell types, including macrophages, the primary target of the Ebola virus. Therefore, while antibodies normally protect the body, this virus is able to use them for faster and easier attachment to target cells (Takada et al. 2003).
In addition being an essential cofactor for the viral RNA polymerase complex, VP35 has been identified as an inhibitor of multiple components of the interferon (IFN) pathways. These are a family of cytokines produced in response to viral infection and regulated by many transcription factors, including interferon regulatory factor 3 (IRF-3). After IRF-3 is stimulated by dsRNA or viral infection, IFN-α/β are secreted. They activate the Janus tyrosine kinase / signal transducer and activator (JAK/STAT) signaling pathway that stimulates transcription of genes encoding antiviral proteins such as protein kinase R (PKR). Upon binding to dsRNA, PKR is activated and phosphorylates translation initiation factor eIF-2, which then arrests protein synthesis and inhibits viral replication (Feng et al. 2007).
VP35 also blocks PKR activation via its C-terminal IRF inhibitory domain, and can even go so far as to reverse PKR phosphorylation (Schümann 2009). FOCUS MORE ON VP35 AND THIS PATH.
As previously mentioned, the second matrix protein, VP24, suppresses interferon production. The binding of IFN-α/β and IFN-γ to their respective receptors activates STAT complexes, a family of transcription factors that regulate immune system gene expression. Normally, STAT1:STAT2 heterodimers and STAT1 homodimers are transported to the nucleus by karyopherin α1 and activate numerous genes involved in antiviral activities. However, VP24 competes with STAT1 to bind karyopherin α1, blocking nuclear accumulation and leading to inhibition of IFN signaling (Reid 2006).
During infection, Ebola controlled macrophages release nitric oxide (NO), a gaseous hormone that normally functions in cell communication. However, in high concentrations, NO depresses the mitochondrial membrane potential, which causes apoptosis in NK and other nearby cells. This is yet another way that Ebola disrupts the immune system (Geisbet et al. 2003).
So far, most of the processes by which Ebola escapes or hampers immune response involve structural proteins on the virus itself. However, one of the most important, and most misunderstood, proteins is the sGP protein that is released by Ebola into the bloodstream. During viral infection, the stability of the vascular endothelium is destroyed by proinflammatory cytokines released from hijacked macrophages or by the cytoxic effects of Ebola replication. However, when sGP is present, there is an apparent recovery of endothelial cell barrier function. While this seems contrary to the actions of other viral proteins, it actually prevents neutrophils and other white blood cells from moving from the blood stream to areas of infection, preventing a coordinated immune response (Wahl-Jensen et al. 2005).
Viral Entry
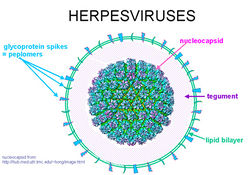
Endocytosis offers an efficient way for viruses to cross the physical barrier of the plasma membrane and move throughout the underlying matrix to cytoplasmic sites suitable for replication. The entry pathway of Ebolavirus must be elucidated if viral pathogenicity is to be understood and drugs are to be developed. After binding to the host cell receptor, the internalized virus is transported through successive endocytic vesicles until it reaches a compartment where conditions favor a conformation shift in viral GP that causes membrane fusion. While there is much evidence suggesting that Ebolavirus enters cells through pH-dependent endocytosis (Schornberg et al. 2006), the specific mechanism has not yet been clearly defined.
Previous studies to clarify the entry have produced conflicting results, with involvement of clathrin-mediated endocytosis (Bhattacharyya et al. 2010), caveolin-mediated endocytosis (Empig & Goldsmith 2002) and a Rho GTPase-dependent pathway that may suggest macropinocytosis all being implicated (Quinn et al. 2009). However, all of these experiments were performed using retrovirus-based pseudotypes in which GP is coated onto the surface of a retrovirus capsid containing a recombinant genome. This removes the need to work in a biosafety level four laboratory, but suffers from non-ideal biochemical characteristics. Only recently have experiments been performed with wild-type Ebolavirus Zaire that demonstrate that cellular entry involves uptake by a macropinocytosis-like mechanism (Saeed et al. 2010).
TALK ABOUT MACROPINOCYTOSIS AND ENTRY.
CONNECTION FROM ENTRY TO TRANSCRIPTION AND REST OF CYCLE.
Filovirus Transcription
During transcription, the RNA genome is transcribed into seven monocistronic mRNAs whose length is determined by highly conserved start and stop signals. These start signals are predicted to form stable stem-loop structures (Sanchez et al. 1993). Just as in other negative sense RNA viruses, the transcription process begins with the binding of the polymerase complex to a single binding site located within the leader region of the genome (Emerson 1982). The complex then slides along the RNA template and sequentially transcribes the individual genes in their 3’ to 5’ order. However, the polymerase is released from the template following mRNA formation, so reinitiation at downstream genes is attenuated. Thus, the first gene, NP, is transcribed at the highest levels, whereas the last gene, L, is transcribed at the lowest (Iverson & Rose 1982).
VP30 is assumed to be a transcription activation factor that is essential for the viral life cycle. While the mechanism is not completely understood, it is suggested that this protein is involved in initiation because VP30-dependent transcription is regulated by RNA of the first transcription start signal. The first 23 nucleotides of this NP mRNA are involved in stem-loop structure formation which might interfere with the progression of transcription by physically hampering polymerase movement (Weik et al. 2002). However, the N-terminus of VP30 contains a Zn+2 binding Cys-His motif (Modrof et al. 2003) and is rich in basic amino acids, thus allowing it to directly interact with RNA (John et al. 2007). Therefore, the protein could either resolve or cover this secondary structure and allow transcription to proceed. Further research has also shown that VP30 is important in transcription reinitiation, and may bind stem-loops formed by the promoter of each Ebolavirus gene (Martínez et al. 2008).
Interestingly, VP24 has also been shown to inhibit transcription and replication of the Ebolavirus genome. While the exact mechanism has not yet been elucidated, it is possible that VP24 binds to NP and hampers the function of VP35, VP30 or L. This interference could be important in converting the viral genome from a transcription or replication competent form to one that is ready for virion assembly and egress (Watanabe et al. 2007).
Viral Budding
While the plasma membrane was initially viewed as a randomly arranged lipid bilayer, it has recently been shown that this cell barrier is much more complex in nature. Certain cholesterol enriched regions known as lipid rafts have been implicated in such diverse functions as channeling external signals into specific second messenger pathways (Cheng et al. 2001) and mediating cell-cell interactions (Moran & Miceli 1998). These processes are possible because these regions concentrate relevant molecules in specialized microenvironments. While these properties are certainly important for cell function, these lipid platforms also serve as a coordination site for the intimate reactions of Ebolavirus proteins required for assembly and budding. This increases the efficiency of virus budding, while lowering the chance that defective, noninfectious particles will be released (Bavari et al. 2002).
These rafts may also impact viral pathogenicity as well as host immune response. Cell signaling molecules normally located in lipid rafts can be incorporated into the envelope of budding virions and used to ensure more efficient replication by affecting biochemical processes of newly infected cells (Bavari et al. 2002). Adhesion proteins could enhance viral entry, while incorporation of certain glycosylphosphatidylinositol (GP1) linked proteins can inhibit the complement pathways CD55 and CD59, which help the virus evade complement-mediated lysis (Saifuddin et al. 1997).
VP40 HAS BEEN IMPLICATED IN VIRAL BUDDING.
Connecting the Cellular Mechanism to the Hemorrhagic Fever – NEEDS WORK
The Ebola virus is normally transmitted by direct contact with infected body fluids or skin/mucus membrane contact. Once inside the body, Ebola first attacks macrophages and monocytes of the immune system. By utilizing antibodies, Ebola is able to bind to or enter these white blood cells (Takada et al. 2003). These infected cells then release large amounts of proinflammatory cytokines that cause increased permeability of the vascular endothelium, as well as facilitating easier entry into endothelial cells. These proteins, like proinflammatory cytokines such as MIP-1 and MCP-1, also recruit more macrophages to the area of the original, which helps maximize the number of cells that Ebola can use to spread throughout the body. These cytokines cannot be cleaned from the bloodstream because hepatocytes in the liver are being destroyed by the virus (Geisbert et al. 2002).
Ebola virions then enter the endothelial cells through interactions between its surface GP and receptors in the lipid raft microdomains (Bavari et al. 2002). They move throughout the cell via microtubules or actin filaments until they begin to undergo transcription and translation (Yonezawa et al. 2005). Some of the products of this process are used to build new virions, while others, like sGP, are used to maximize the time Ebola can use a cell before its death (Simmons et al. 2002). During this process, Ebola GP is causing the cell to detach from those around it via interactions with VE-cadherin, as well as lose contact with its basement membrane thanks to interactions with integrin and CAMs (Wahl-Jensen et al. 2005). The newly created particles leave via lipid rafts. Due to this process, the vascular endothelium of the body is destabilized, which leads to the massive blood loss, which causes or relates to many of the symptoms, that is characteristic of Ebola patients (Bavari et al. 2002, Fig. 4).
During this process, the immune system is going haywire. Interferons are not being made (Basler et al. 2003). T cells cannot recognize that there is a problem (Sullivan et al. 2005). White blood cells cannot get to their necessary location because sGP prevents their movement (Wahl-Jensen et al. 2005). Macrophages and monocytes are releasing massive amounts of proinflammatory cytokines that are aiding in the destruction of the vascular endothelium, while also causing activation of the coagulation cascade. This cascade activates fibrinogenic and fibrinolytic pathways. Fibrin is a protein that is involved in the clotting of blood, so these pathways involve the building up or breaking down of fibrin meshes that form blood clots. These pathways eventually lead to disseminated intravascular coagulation, a state where the blood starts to coagulate around the body and use up platelets and coagulating factors. This puts the body in a paradoxical state where the organism can either die of hypovolemic shock from massive hemorrhage, or from catastrophic thrombosis, the formation of blood clots around the body (Geisbert et al. 2003).
References
Basler, C.F., Wang, X., Mühlberger, E., Volchkov, V., Paragas, J., Klenk, H.D., Garcia-Sastre, A. & Palese, P. (2000). The Ebola virus VP35 protein functions as a type I IFN antagonist. PNAS, 97, 12289-12294.
Basler, C.F., Mikulasova, A., Martinez-Sobrido, L., Paragas, J., Mühlberger, E., Bray, M., Klenk, H.D., Palese, P. & García-Sastre, A. (2003). The Ebola Virus VP35 Protein Inhibits Activation of Interferon Regulatory Factor 3. Journal of Virology, 77(14), 7945-7956.
Bavari, S., Bosio, C.M., Wiegand, E., Ruthel, G., Will, A.B., Geisbert, T.W., Hevey, M., Schmaljohn, C., Schmaljohn, A. & Aman, M.J. (2002). Lipid Raft Microdomains: A Gateway for Compartmentalized Trafficking of Ebola and Marburg Viruses. The Journal of Experimental Medicine, 195(5), 593-602.
Bhattacharyya, S., Warfield, K.L., Ruthel, G., Bavari, S., Aman, M.J. & Hope, T.J. (2010). Ebola virus uses clathrin-mediated endocytosis as an entry pathway. Virology, 401, 18-28.
Borio, L., Inglesby, T., Peters, C.J., Schmaljohn, A.L., Hughes, J.M., Jahrling, P.B., Ksiazek, T., Johnson, K.M., Meyerhoff, A., O’Toole, T., Ascher, M.S., Bartlett, J., Breman, J.G., Eitzen, Jr., E.M., Hamburg, M., Hauer, J., Henderson, D.A., Johnson, R.T., Kwik, G., Layton, M., Lillibridge, S., Nabel, G.J., Osterholm, M.T., Perl, T.M., Russell, P. & Tonat, K. (2002). Hemorrhagic fever viruses as biological weapons: medical and public health management. Journal of the American Medical Association, 287, 2391-2405.
CDC Special Pathogens Branch. (2010). Ebola Hemorrhagic Fever Case Count and Location List. Ebola Hemorrhagic Fever Information Packet.
Cheng, P.C., Cherukuri, A., Dykstra, M., Malapati, S., Sproul, T., Chen, M.R. & Pierce, S.K. (2001). Floating the raft hypothesis: the roles of lipid rafts in B cell antigen receptor function. Semininars in Immunology, 13, 107-114.
Crary, S.M., Towner, J.S., Honig, J.E., Shoemaker, T.R. & Nichol, S.T. (2003). Analysis of the role of predicted RNA secondary structures in Ebola virus replication. Virology, 306(2), 210-218.
Emerson, S.U. (1982). Reconstitution studies detect a single polymerase entry site on the vesicular stomatitis virus genome. Cell, 31, 635-642.
Empig, C.J. & Goldsmith, M.A. (2002). Association of the caveola vesicular system with cellular entry by filoviruses. Journal of Virology, 76, 5266-5270.
Feldmann, H., Klenk, H.D. & Sanchez, A. (1993). Molecular biology and evolution of filoviruses. Archives of Virology, Supplementum, 7, 81-100.
Geisbert, T., Hensley, L., Larsen, T., Young, H., Reed, D., Geisbert, J., Scott, D., Kagan, E., Jahrling, P. & Davis, K. (2003). Pathogenesis of Ebola Hemorrhagic Fever in Cynomolgus Macaques. American Journal of Pathology, 163, 2347-2370.
Hoenen, T., Groseth, A., Kolesnikova, L., Theriault, S., Ebihara, H., Hartlieb, B., Bamberg, S., Feldmann, H., Ströher, U. & Becker, S. (2006). Infection of Naïve Target Cells with Virus-Like Particles: Implications for the Function of Ebola Virus VP24. Journal of Virology, 80(14), 7260-7264.
Huang, Y., Xu, L., Sun, Y. & Nabel, G.J. (2002). The assembly of Ebola virus nucleocapsid requires virion-associated proteins 35 and 24 and posttranslational modification of nucleoprotein. Molecular Cell, 10, 307-316.
Iverson, L.E. & Rose, J.K. (1982). Sequential synthesis of 5’-proximal vesicular stomatitis virus mRNA sequences. Journal of Virology, 44, 356-365.
Jasenosky, L.D., Neumann, G., Lukashevich, I. & Kawaoka, Y. (2001). Ebola Virus VP40-Induced Particle Formatin and Association with the Lipid BIlayer. Journal of Virology, 75(11), 5205-5214.
John, S.P., Wang, T., Steffen, S., Longhi, S., Schmaljohn, C.S. & Jonsson, C.B. (2007). Ebola Virus VP30 Is an RNA Binding Protein. Journal of Virology, 81(17), 8967-8976.
Johnson, K.M. & Breman, J.G. (1978) Ebola haemorrhagic fever in Zaire, 1976. Bulletin of the World Health Organization, 56(2), 271-293.
Klenk, H.-D. & Feldmann, H. (2004). Ebola and Marburg Viruses: Molecular and Cellular Biology. Wymondham: Horizon Bioscience.
Martínez, M.J., Biedenkopf, N., Volchkova, V., Hartlieb, B., Alazard-Dany, N., Reynard, O., Becker, S. & Volchkov, V. (2008). Role of Ebola Virus VP30 in Transcription Reinitiation. Journal of Virology, 82(24), 12569-12573.
Modrof, J., Becker, S. & Muhlberger, E. (2003). Ebola virus transcription activator VP30 is a zinc-binding protein. Journal of Virology, 77, 3334-3338.
Moran, M. & Miceli, M.C. (1998). Engagement of GPI-linked CD48 contributes to TCR signals and cytoskeletal reorganization: a role for lipid rafts in T cell activation. Immunity, 9, 787-796.
Mühlberger, E.,Weik, M., Volchkov, V.E., Klenk, H.D. & Becker, S. (1999). Comparison of the transcription and replication strategies of Marburg virus and Ebola virus by using artificial replication systems. Journal of Virology, 73, 2333-2342.
Pratt, W.D., Wang, D., Nichols, D.K., Luo, M., Woraratanadharm, J., Dye, J.M., Holman, D.H. & Dong, J.Y. (2010). Protection of Nonhuman Primates against Two Species of Ebola Virus Infection with a Single Complex Adenovirus Vector. Clinical and Vaccine Immunology, 17(4), 572-581.
Quinn, K., Brindley, M.A., Weller, M.L., Kaludov, N., Kondratowicz, A., Hunt, C.L., Sinn, P.L., McCray, P.B., Stein, C.S., Davidson, B.L., Flick, R., Mandell, R., Staplin, W., Maury, W. & Chiorini, J.A. (2009). Rho GTPases Modulate Entry of Ebola Virus and Vesicular Stomatitis Virus Pseudotyped Vectors. Journal of Virology, 83(19), 10176-10186.
Reid, S.P., Leung, L.W., Hartman, A.L., Martinez, O., Shaw, M.L., Carbonnelle, C., Volchkov, V.E., Nichol, S.T. & Basler, C.F. (2006). Ebola Virus VP24 Binds Karyopherin α1 and Blocks STAT1 Nuclear Accumulation. Journal of Virology, 80(11), 5156-5167.
Saeed, M.F., Kolokoltsov, A.A., Albrecht, T. & Davey, R.A. (2010). Cellular Entry of Ebola Virus Involves Uptake by a Macropinocytosis-Like Mechanism and Subsequent Trafficking through Early and Late Endosomes. PLOS Pathogens, 6(9).
Saifuddin, M., Hedayati, T., Atkinson, J.P., Holguin, M.H., Parker, C.J. & Spear, G.T. (1997). Human immunodeficiency virus type 1 incorporates both glycosyl phosphatidylinositol-anchored CD55 and CD59 and integral membrane CD46 at levels that protect from complement-mediated destruction. Journal of General Virology, 78, 1907-1911.
Sanchez, A., Kiley, M.P., Holloway, B.P. & Auperin, D.D. (1993). Sequence analysis of the Ebola virus genome: organization, genetic elements, and comparison with the genome of Marburg virus. Virus Research, 29, 215-240.
Schümann, M., Gantke, T. & Mühlberger, E. (2009). Ebola Virus VP35 Antagonizes PKR Activity through Its C-Terminal Interferon Inhibitory Domain. Journal of Virology, 83(17), 8993-8997.
Schornberg, K., Matsuyama, S., Kabsch, K., Delos, S., Bouton, A. & White, J. (2006). Role of endosomal cathepsins in entry mediated by the Ebola virus glycoprotein. Journal of Virology, 80(8), 4174-4178.
Simmons, G., Wool-Lewis, R., Baribaud, F., Netter, R. & Bates, P. (2002). Ebola Virus Glycoproteins Induce Global Surface Protein Down-Modulation and Loss of Cell Adherence. Journal of Virology, 76(5), 2518-2528.
Sullivan, N., Peterson, M., Yang, Z., Kong, W., Duckers, H., Nabel, E. & Nabel, G. (2005). Ebola Virus Glycoprotein Toxicity Is Mediated by a Dynamin-Dependent Protein-Trafficking Pathway. Journal of Virology, 79(1), 547-553.
Takada, A., Feldmann, H., Ksiazek, T. & Kawaoka, Y. (2003). Antibody-Dependent Enhancement of Ebola Virus Infection. Journal of Virology, 77(13), 7539–7544.
Volchkov, V.E., Becker, S., Vochkova, V.A., Ternovoj, V.A., Kotov, A.N., Netesov, S.V. & Klenk, H.D. (1995). GP mRNA of Ebola virus is edited by the Ebola virus polymerase and by T7 and vaccinia virus polymerases. Virology, 214, 421-430.
Wahl-Jensen, V., Afanasieva, T., Seebach, J., Ströher, U., Feldmann, H. & Schnittler, H. (2005). Effects of Ebola Virus Glycoproteins on Endothelial Cell Activation and Barrier Function. Journal of Virology, 79(16), 10442-10450.
Watanabe, S., Noda, T., Halfmann, P., Jasenosky, L. & Kawaoka, Y. (2007). Ebola Virus (EBOV) VP24 Inhibits Transcription and Replication of the EBOV Genome. The Journal of Infectious Diseases, 196, S284-290.
Weik, M., Modrof, J., Klenk, H.D., Becker, S. & Mühlberger, E. (2002). Ebola Virus VP30-Mediated Transcription Is Regulated by RNA Secondary Structure Formation. Journal of Virology, 76(17), 8532-8539.
World Health Organization. (2010). Ebola Hemorrhagic Fever. Epidemic and Pandemic Alert and Response (EPR).
Yonezawa, A., Cavrois, M. & Greene, W.C. (2005). Studies of Ebola Virus Glycoprotein-Mediated Entry and Fusion by Using Pseudotyped Human Immunodeficiency Virus Type 1 Virions: Involvement of Cytoskeletal Proteins and Enhancement by Tumor Necrosis Factor Alpha. Journal of Virology, 79(2), 918-926.
Page authored for BIOL 375 Virology, September 2010