Geomicrobiology: Difference between revisions
m (Added Bioremediation link) |
(Copied over the Elemental Cycles (Without images); Minor reorganization of Space Exploration section) |
||
Line 39: | Line 39: | ||
===Mineral Cycles Involving Microbes=== | ===Mineral Cycles Involving Microbes=== | ||
Microbes are involved in and drive many geochemical cycles. Among them, [https://microbewiki.kenyon.edu/index.php/Nitrogen_Cycle the nitrogen cycle], the phosphorus cycle, the sulfur cycle, and the iron cycle. | |||
====Phosphorus Cycle==== | ====Phosphorus Cycle==== | ||
Phosphorus is an important nutrient for all living organisms. Most phosphorus found in living systems are in the form of inorganic phosphate. On Earth virtually all known phosphorus exists in the +5 oxidation state. Nonetheless, there are also two additional known forms: phosphonates (+3) and phosphonates (+1). Microbes immobilize, mineralize, and solubilize phosphorus. | |||
The phosphorus cycle does not include a gas phase, and thus very little phosphorus makes its way into the atmosphere (small amounts of phosphoric acid can make their way into the atmosphere to contribute to acid rain). The phosphorus cycle begins in sedimentary rock and is released into the soil and water through weathering. Microbes (and plants, as well) assist in the solubilization of mineral phosphorus in soil through the production of acids to lower the soil pH, as well as the secretion of chelating agents to bind the metal ions that would bind to the phosphate (Silva et al, pg 472). These soluble phosphates are immobilized by living organisms, and can be produced through mineralization reactions. | |||
<i>Actinomycetes</i>, <i>Pseudomonas</i>, and <i>Bacillus</i> species are among the bacteria that solubilize inorganic phosphorus. <i>Aspergillus</i> and <i>Penicillium</i> are among the fungi that solubilize phosphorus. Additionally in the rhizosphere, mycorrhizal symbioses between fungi and plants greatly increase phosphorus uptake by plants. Some of the fungi involved are <i>Zygomycetes</i> in the order of <i>Mucorales</i> and <i>Ascomycetes</i> (Metcalf et al., 1998 and Rosling et al., 2007). | |||
Microbes also increase the mineralization process of phosphorous in soil by using phosphatase enzymes to hydrolyze organic phosphorus and convert it back to its inorganic form (Richardson and Simpson 2011). | |||
====Sulfur Cycle==== | ====Sulfur Cycle==== | ||
Most sulfur is contained in rock, with small amounts in the atmosphere and water. In soil, however, most sulfur is organic, as part of an organism; the addition of new sulfur into the ecosystem is through the weathering of sulfur-containing stone. Thus, the sulfur cycle in soil is driven by microorganisms. Some of the microbially-induced transformations sulfur undergoes are: reduction and oxidation, mineralization and immobilization, and volatilization. In short, sulfur bacteria convert mineral sulfur into soluble forms, which are then taken up into microbial biomass, or converted into other mineral forms. Sulfur exits the soil through leaching or volatilization of sulfur. | |||
As a biochemical, sulfur is important in that it has a wide range of stable redox states and as a carbon carrier. Inorganic sulfur can be used as a terminal electron acceptor for energy. Sulfate-reducing bacteria are comprised of several groups of bacteria that use sulfate as an oxidizing agent, reducing it to sulfide. Most sulfate-reducing bacteria can also use other oxidized sulfur compounds such as sulfite and thiosulfate, or elemental sulfur. This type of metabolism is called dissimilatory, since sulfur is not incorporated or assimilated into any organic compounds (Campbell et al., 2006). | |||
Anaerobic sulfur reducing bacteria reduce sulfate ions to hydrogen sulfide. These sulfur reducing bacteria are heterotrophic organisms that use sulfur ions as terminal electron acceptors in their metabolism. These organisms include bacteria of the genera <i>Desulfovibrio</i> and <i>Desulfotomaculum</i>. | |||
The bacterial genera <i>Thiobacillus</i>, <i>Sulfolobus</i>, and <i>Thiomicrospira</i> oxidize sulfur to produce sulfuric acid as a byproduct. These bacteria accelerate the generation of [http://technology.infomine.com/enviromine/ard/Microorganisms/roleof.htm acid rock drainage] (ARD) from pyritic and pyrrhotitic rocks. <i>Acidithiobacillus thiooxidans</i> also produces sulfuric acid and, in conjunction with others of the same genus, is currently used in a mining technique called bioleaching whereby metals are extracted from their ores through oxidation. The bacteria are used as catalysts in the oxidation reaction. Sulfate-reducing bacteria have been considered for remediating water contamination produced by other bacteria from acid mine tailings. | |||
====Iron Cycle==== | ====Iron Cycle==== | ||
After oxygen, iron is the most abundant redox-active element in the Earth’s crust. However, it is not easily available in its preferred state. The availability of iron depends on the aerobic condition and pH level of the soil. Under aerobic conditions, iron readily binds with oxygen, forming insoluble (and therefore non-bioavailable) iron oxides. However, at low pH, both common forms of Fe (II, III) are readily soluble. In terms of mineralization and solubilization, microbes accomplish both through redox reactions (Colombo et al. 2014). Iron can be mineralized, immobilized, oxidized, and reduced. | |||
Fe(III) is an important electron acceptor in organic matter decomposition in saturated soil. As this form of iron is soluble, the iron leaves the soil; this causes gleying of the soil (the color of the soil becomes grey or blue), as the color of the sand’s sand content becomes dominant. Fe(III) reduction also corrodes steel which causes many industrial problems. One of the most common iron redox reactions in anaerobic soils is the mineralization of iron through binding sulfur to iron, producing pyrite (Colombo et al. 2014). Furthermore, in these flooded conditions, iron is also used as an energy source; anaerobic iron-reducing bacteria use iron as an terminal electron acceptor in respiration (Lovley et al 2004). | |||
Siderophores are iron transporting ligands that are produced by bacteria and fungi that reduce Fe(III) to Fe(II). When microbes experience iron deficiency, siderophores are secreted into the environment to capture iron. The solubilized iron is then taken up by the microbe through a siderophore receptor protein on the outer cell membrane. Iron is the only known essential element for which these specific organic shuttles operate. | |||
==Examples of Geomicrobiology Interactions in nature== | ==Examples of Geomicrobiology Interactions in nature== | ||
Line 58: | Line 81: | ||
====Life Support and Waste Management==== | ====Life Support and Waste Management==== | ||
[[Image:Melissaloop.jpeg|thumb|300px|right|General concept of the MELISSA loop for advanced life support [http://ecls.esa.int/ecls/?p=newmelissaloop Image Source]]] | [[Image:Melissaloop.jpeg|thumb|300px|right|General concept of the MELISSA loop for advanced life support [http://ecls.esa.int/ecls/?p=newmelissaloop Image Source]]] | ||
The biggest use of microbes in space exploration and settlement would be the use of photosynthetic organisms to convert water and carbon dioxide into oxygen and food in what is known as a biological life support system. Additionally the waste from these processes could be used to make more carbon dioxide, recycling the nutrients, water and carbon. The Micro Ecological Life Support System, designed by European Space Agency, is a model system of an advance biological life support system that would be based on different species of microbes and plants. Consisting of many bioreactors and plant chambers, MELiSSA would break down the organic waste from the crew and non-edible parts of the higher plants (Gòdia et al 2002). We can learn a lot about how MELiSSA works by looking at soil systems and processes here on Earth. The MELiSSA loop is the basis of the life support system created by the ESA and uses processes we see all the time on earth. | The biggest use of microbes in space exploration and settlement would be the use of photosynthetic organisms to convert water and carbon dioxide into oxygen and food in what is known as a biological life support system. Additionally the waste from these processes could be used to make more carbon dioxide, recycling the nutrients, water and carbon. The Micro Ecological Life Support System Alternative (MELiSSA), designed by European Space Agency, is a model system of an advance biological life support system that would be based on different species of microbes and plants. Consisting of many bioreactors and plant chambers, MELiSSA would break down the organic waste from the crew and non-edible parts of the higher plants (Gòdia et al 2002). We can learn a lot about how MELiSSA works by looking at soil systems and processes here on Earth. | ||
The MELiSSA loop is the basis of the life support system created by the ESA and uses processes we see all the time on earth. For example, the transformations of nitrogen (mineralization, nitrification, immobilization and denitrification) show the different ways nitrogen is transformed in soil and in the atmosphere. From studying how microbes interact in soil has helped us create MELiSSA, which combines multiple systems to produce food, water and oxygen for space explorers. | |||
====Energy Production==== | ====Energy Production==== | ||
One example of the use of microbes in in the production of energy and additional waste management is the use of a MFC, or microbial fuel cell. MFCs can be used to generate electricity and process waste, but they currently produce low amounts of energy and depend on microbes processing organic matter in an anaerobic anode chamber (Cockell 2010). On earth MFCs can be made using the soil found all around us because soil contains a diverse population of microbes, along with the nutrients needed by those microbes to generate energy. | One example of the use of microbes in in the production of energy and additional waste management is the use of a MFC, or microbial fuel cell. MFCs can be used to generate electricity and process waste, but they currently produce low amounts of energy and depend on microbes processing organic matter in an anaerobic anode chamber (Cockell 2010). On earth MFCs can be made using the soil found all around us because soil contains a diverse population of microbes, along with the nutrients needed by those microbes to generate energy. |
Revision as of 01:56, 15 March 2016
Geomicrobiology is the interdisciplinary study of the interactions of microorganisms with earth materials. It concerns the role of microbes in geological and geochemical processes. The field of geomicrobiology has revealed new insights into the intersection of life with the physical and chemical composition of Earth’s surface. Soil microbes play a large role in the transformations of elements and minerals. The interactions between microbes and elements and minerals is especially important in the near surface environment known as Earth’s Critical Zone, where biotic and abiotic factors regulate the conditions for life-sustaining resources. Virtually all elements can be transformed by microbes and many elemental cycles depend on soil microbes (Gadd 2010). The relationships between microorganisms and these inorganic compounds have important implications for both the surrounding natural environment and human use. Soil microbes can be used by humans in mineral resource extraction and bioremediation.
Key processes of geomicrobiology
Geomicrobiological processes are relevant in many natural environments including aquifers, geological and geochemical processes, extreme environments (acidic, extreme temperatures and saline conditions) and metal ion reduction.
Weathering
Weathering plays an important role on Earth ecosystems and is important for the release of nutrients into the biosphere as a result of rock dissolution or the regulation of long-term climate by the consumption of atmospheric carbon dioxide from silicate alterations. Weathering has been considered a strictly physical and chemical process. Nonetheless, many weathering processes can be affected by the presence of microbial communities. All types of rocks are susceptible to microbial weathering, including siliceous and calcareous rocks. In general microbial weathering is due to the formation of organic acids or the production of metal-chelating siderophores on the surface of rock or minerals. Other processes include oxidative or reductive conditions of metals on rocks or minerals (Herrera et al., 2008).
Precipitation of carbonates and phosphates
The influence of bacteria in mineral precipitation has been described in different natural habitats including aquatic environments and geological systems. Carbonate precipitation is controlled by bacterial processes and one of the mechanisms proposed is the production of ammonium by metabolizing nitrogen base organic compounds which increases environment pH. Other authors report that the matrix of extracellular polymeric secretions (EPS) affects mineral precipitation Simultaneous precipitation of struvite (MgNH4PO4•6H2O) associated with bacterial activity has been described in vitro carbonate precipitation experiments as well as carbonate and phosphate precipitation by bacteria from saline soils (Delgado et al., 2008).
Examples of microbial metabolism of Minerals and inorganic substances
Table of Microbial Roles for Representative Elements (Taken from Gadd 2010) | |
---|---|
Elements | Microbial roles in elemental cycles |
Phosphorus (P) | Dissolution of inorganic phosphate minerals in soils; Decomposition of organic phosphorus; Release of organically bound phosphorus by use of phosphatase enzymes; Assimilation and transformation of inorganic phosphorus species; Transformations of soil organic phosphorus; Phosphorus transfer to plants by mycorrhizae |
Sulfur (S) | Degradation of organic sulfur compounds; Sulfur transformations; Assimilation of organic and inorganic sulfur; Sulfidogenesis, Sulfur accumulation, SO42- reduction and assimilation; Oxidation of H2S to S(0) and reduction of S(0) to H2S |
Iron (Fe) | Bioweathering of iron-containing minerals in soil; Iron solubilization by siderophores; Fe(III) reduction to Fe(II); Fe(II) oxidation to Fe(III); Iron biomineralization to oxides, hydroxides, carbonates, sulfides, etc. Metal sorption to iron oxides |
Manganese (Mn) | Mn(II) oxidation and immobilization as Mn(IV) oxides; Bioaccumulation of Manganese oxides to surfaces and exopolymers; contribution to desert varnish formation; biosorption; accumulation; intracellular precipitation; biomineralization; metal sorption to manganese oxides |
Magnesium (Mg), Calcium (Ca), Nickel (Ni), Zinc (Zn), Cadmium (Cd), and Strontium (Sr) | Bioweathering of minerals in soil; biosorption; uptake and accumulation; bioprecipitation of oxalates, sulfides, carbonate, phosphates, etc. |
Copper (Cu) | Mobilization from copper containing minerals in soils; CuS formation; biosorption; uptake and accumulation; bioprecipitation |
Mercury (Hg) | Biomethylation; Reduction of Hg(III) to Hg(0); Oxidation of Hg(0) to Hg(II). Mercury volatilization as Hg(0); degradation of organomercurials; biosorption; accumulation |
Selenium (Se) | Reductive transformation of Se (VI) to Se(IV) to Se(0); Se(0) oxidation; Biomethylation and demethylation; assimilation of organic and inorganic Se compounds |
Mineral Cycles Involving Microbes
Microbes are involved in and drive many geochemical cycles. Among them, the nitrogen cycle, the phosphorus cycle, the sulfur cycle, and the iron cycle.
Phosphorus Cycle
Phosphorus is an important nutrient for all living organisms. Most phosphorus found in living systems are in the form of inorganic phosphate. On Earth virtually all known phosphorus exists in the +5 oxidation state. Nonetheless, there are also two additional known forms: phosphonates (+3) and phosphonates (+1). Microbes immobilize, mineralize, and solubilize phosphorus.
The phosphorus cycle does not include a gas phase, and thus very little phosphorus makes its way into the atmosphere (small amounts of phosphoric acid can make their way into the atmosphere to contribute to acid rain). The phosphorus cycle begins in sedimentary rock and is released into the soil and water through weathering. Microbes (and plants, as well) assist in the solubilization of mineral phosphorus in soil through the production of acids to lower the soil pH, as well as the secretion of chelating agents to bind the metal ions that would bind to the phosphate (Silva et al, pg 472). These soluble phosphates are immobilized by living organisms, and can be produced through mineralization reactions.
Actinomycetes, Pseudomonas, and Bacillus species are among the bacteria that solubilize inorganic phosphorus. Aspergillus and Penicillium are among the fungi that solubilize phosphorus. Additionally in the rhizosphere, mycorrhizal symbioses between fungi and plants greatly increase phosphorus uptake by plants. Some of the fungi involved are Zygomycetes in the order of Mucorales and Ascomycetes (Metcalf et al., 1998 and Rosling et al., 2007).
Microbes also increase the mineralization process of phosphorous in soil by using phosphatase enzymes to hydrolyze organic phosphorus and convert it back to its inorganic form (Richardson and Simpson 2011).
Sulfur Cycle
Most sulfur is contained in rock, with small amounts in the atmosphere and water. In soil, however, most sulfur is organic, as part of an organism; the addition of new sulfur into the ecosystem is through the weathering of sulfur-containing stone. Thus, the sulfur cycle in soil is driven by microorganisms. Some of the microbially-induced transformations sulfur undergoes are: reduction and oxidation, mineralization and immobilization, and volatilization. In short, sulfur bacteria convert mineral sulfur into soluble forms, which are then taken up into microbial biomass, or converted into other mineral forms. Sulfur exits the soil through leaching or volatilization of sulfur.
As a biochemical, sulfur is important in that it has a wide range of stable redox states and as a carbon carrier. Inorganic sulfur can be used as a terminal electron acceptor for energy. Sulfate-reducing bacteria are comprised of several groups of bacteria that use sulfate as an oxidizing agent, reducing it to sulfide. Most sulfate-reducing bacteria can also use other oxidized sulfur compounds such as sulfite and thiosulfate, or elemental sulfur. This type of metabolism is called dissimilatory, since sulfur is not incorporated or assimilated into any organic compounds (Campbell et al., 2006).
Anaerobic sulfur reducing bacteria reduce sulfate ions to hydrogen sulfide. These sulfur reducing bacteria are heterotrophic organisms that use sulfur ions as terminal electron acceptors in their metabolism. These organisms include bacteria of the genera Desulfovibrio and Desulfotomaculum.
The bacterial genera Thiobacillus, Sulfolobus, and Thiomicrospira oxidize sulfur to produce sulfuric acid as a byproduct. These bacteria accelerate the generation of acid rock drainage (ARD) from pyritic and pyrrhotitic rocks. Acidithiobacillus thiooxidans also produces sulfuric acid and, in conjunction with others of the same genus, is currently used in a mining technique called bioleaching whereby metals are extracted from their ores through oxidation. The bacteria are used as catalysts in the oxidation reaction. Sulfate-reducing bacteria have been considered for remediating water contamination produced by other bacteria from acid mine tailings.
Iron Cycle
After oxygen, iron is the most abundant redox-active element in the Earth’s crust. However, it is not easily available in its preferred state. The availability of iron depends on the aerobic condition and pH level of the soil. Under aerobic conditions, iron readily binds with oxygen, forming insoluble (and therefore non-bioavailable) iron oxides. However, at low pH, both common forms of Fe (II, III) are readily soluble. In terms of mineralization and solubilization, microbes accomplish both through redox reactions (Colombo et al. 2014). Iron can be mineralized, immobilized, oxidized, and reduced.
Fe(III) is an important electron acceptor in organic matter decomposition in saturated soil. As this form of iron is soluble, the iron leaves the soil; this causes gleying of the soil (the color of the soil becomes grey or blue), as the color of the sand’s sand content becomes dominant. Fe(III) reduction also corrodes steel which causes many industrial problems. One of the most common iron redox reactions in anaerobic soils is the mineralization of iron through binding sulfur to iron, producing pyrite (Colombo et al. 2014). Furthermore, in these flooded conditions, iron is also used as an energy source; anaerobic iron-reducing bacteria use iron as an terminal electron acceptor in respiration (Lovley et al 2004).
Siderophores are iron transporting ligands that are produced by bacteria and fungi that reduce Fe(III) to Fe(II). When microbes experience iron deficiency, siderophores are secreted into the environment to capture iron. The solubilized iron is then taken up by the microbe through a siderophore receptor protein on the outer cell membrane. Iron is the only known essential element for which these specific organic shuttles operate.
Examples of Geomicrobiology Interactions in nature
Rhizosphere
Volcanic Hot Springs
Examples of Applied Geomicrobiology
Geomicrobiology has many useful applications for humans. Many microbes are involved with breaking down toxic compounds (see Bioremediation), mineral extraction, and the manufacturing of valuable minerals and compounds.
Space exploration
Geomicrobiology is being considered an important tool in the exploration and settlement of space because microorganisms can be used for a variety of uses, such as life support and waste management, energy production, extraction of industrially useful minerals, and the search for life using microbe-mineral interactions. Below are a couple of examples of how humans can use processes that are studied on earth to help explore and colonize space.
Life Support and Waste Management
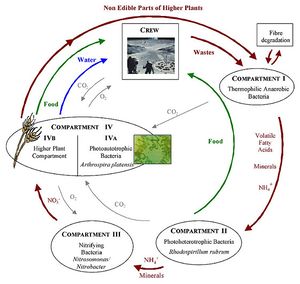
The biggest use of microbes in space exploration and settlement would be the use of photosynthetic organisms to convert water and carbon dioxide into oxygen and food in what is known as a biological life support system. Additionally the waste from these processes could be used to make more carbon dioxide, recycling the nutrients, water and carbon. The Micro Ecological Life Support System Alternative (MELiSSA), designed by European Space Agency, is a model system of an advance biological life support system that would be based on different species of microbes and plants. Consisting of many bioreactors and plant chambers, MELiSSA would break down the organic waste from the crew and non-edible parts of the higher plants (Gòdia et al 2002). We can learn a lot about how MELiSSA works by looking at soil systems and processes here on Earth.
The MELiSSA loop is the basis of the life support system created by the ESA and uses processes we see all the time on earth. For example, the transformations of nitrogen (mineralization, nitrification, immobilization and denitrification) show the different ways nitrogen is transformed in soil and in the atmosphere. From studying how microbes interact in soil has helped us create MELiSSA, which combines multiple systems to produce food, water and oxygen for space explorers.
Energy Production
One example of the use of microbes in in the production of energy and additional waste management is the use of a MFC, or microbial fuel cell. MFCs can be used to generate electricity and process waste, but they currently produce low amounts of energy and depend on microbes processing organic matter in an anaerobic anode chamber (Cockell 2010). On earth MFCs can be made using the soil found all around us because soil contains a diverse population of microbes, along with the nutrients needed by those microbes to generate energy.
Microbial Mineral Resource Extraction
Gold Production, Detection, and Transformation by Microbes
Role of Geomicrobiology in Bioleaching
Role of Magnetotactic Bacteria
Current Research and Links
There is a very interesting site hosted by the Geomicrobiology & Environmental Microbiology Studies at Louisiana State University [1] This site is filled with current research photos and informative discussions concerning the topic.
Geomicrobiology of the Tinto River, a model of interest for biohydrometallurgy Researchers have been using molecular and non molecular techniques to study the microbial ecology in the Tinto river (Southwestern Spain). The acidic conditions of the river (average pH 2.3) make it an example of an extreme environment. Researchers have found while sulfur does play a role in the rivers acidity Iron is the key element. Within the river Iron acts both as an substrate for oxidizing bacteria and as an electron acceptor in the anoxic areas of the river. Isotopic dating has concluded the rivers conditions have evolved from natural phenomena and are not the results of manmade pollutants. [2]
Key Laboratory of Marine Geology and Environment, Institute of Oceanology, China; has published on current research showing Rhodobaterales are primary surface colonizers in temperate costal marine waters. [3] In there study used nonnutritive inert materials such as plexi glass and glass to test for microorgansis in seawater off the Qingdao coast. The understanding is bacteria are know to be surface colonizers but which bacteria was relativly unknown untill now.
References
Campbell, B.J., Engel, A.S., Porter, M.L., and Takai, K. (2006) The versatile Epsilonproteobacteria: Key players in sulphidic habitats. Nature Reviews Microbiology. 4: 458-468. [4]
Dang, H., Li, T., Chen, M., Hunng, G. 2007.ross-Ocean Distribution of Rhodobacterales Bacteria as Primary Surface Colonizers in Temperate Coastal Marine Waters. Applied and Environmental Microbiology. 70:1 52-60.
Delgado, G. Delgado, R., Parraga, J., Rivadeneyra, M. A., Aranda, V. 2008. Precipitation of Carbonates and Phosphates by Bacteria in Extract Solutions from a Semi-arid Saline Soil. Influence of Ca2+ and Mg2+ Concentrat ions and Mg2+/Ca2+ Molar Ratio in Biomineralization. Geomicro. J. 25:1–13.
Engelen, B., Ziegelmuller, K., Wolf, L., Kopke, B., Gittel, A., Cypionka, H., Treude, T., Nakagawa, S., Inahaki, F., Lever, M. A., Steinsbu, B. O. 2008. Fluids from the Oceanic Crust Support Microbial Activities within the Deep Biosphere. Geomicro. J. 25:56-66.
Fomina, M., Podgorsky, V. S., Olishevska, V., Kadoshnikov, V. M., Pisanska, I. R., Hillier, S., Gadd, G. M. 2007. Fungal Deterioration of Barrier Concrete used in Nuclear Waste Disposal. Geomicro. J. 24:643-653.
Gonzalez-Toril, E., Gomez, F.,Rodriquez, N., Fernandez, D., Zuluaga, J., Marin, I., and Amils, R. "Geomicrobiology of the Tinto River, a model of interest for biohydrometallurgy". Hydrometalluragy. 71:301-309.
Herrera, A., Cockell, C. S., Self, S., Blaxter, M., Reitner, J., Arp, G., Drose, W., Thorsteinsson, T. , Tindle, A. G.2008. Bacterial Colonization and Weathering of Terrestrial Obsidian in Iceland. Geomicro. J. 25: 25-37.
Metcalf, W. W., Wolfe, R. S. 1998. Molecular Genetic Analysis of Phosphite and Hypophosphite Oxidation by Pseudomonas stutzeri WM88. J. Bacter. 21: 5547-5558
Murray, K. J., Tebo, B. M. 2007. Cr(III) Is Indirectly Oxidized by the Mn(II)-Oxidizing Bacterium Bacillus sp. Strain SG-1 Environ. Science Technology. 41: 528-533.
Rosling, A., Suttle, K B., Johansson, E., Van Hees, P. A. W., Banfield, J. F. 2007. Phosphorous availability influences the dissolution of apatite by soil fungi. Geobiology 5 (3): 265–280.
Senko, J. M., Suflita, J. M., Krumholz, L. R. 2005. Geochemical Controls on Microbial Nitrate-Dependent U(IV) Oxidation. Geomicro. J. 22:371-378
Sylvia, D. M., Hartel, P. G., Fuhrmann, J. J., Zuberer, D. A. Principles of Applications of Soil Microbiology. 2nd edition. 2005. Pearson Prentice Hall.
Edited by student of Kate Scow