Rose Rosette Virus
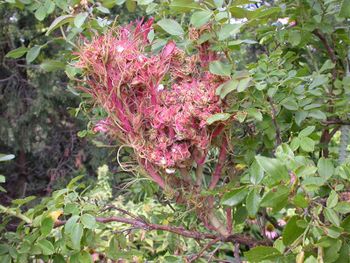
Introduction
By Jacob Scharfetter
Rose rosette virus (RRV), also known as Rose rosette disease (RRD), is a viral plant pathogen. The symptoms of Rose Rosette Virus (RRV) was first recognized and recorded in Canada 77 years and has since become one of the most destructive diseases of commercial roses[1][2]. The plant pathogen RRV has only been found to affect the genus Rosa[3]. Most Rosa spp. are susceptible to RRV, making RRV a significant problem for landscapers and horticulturalists[4]. However, non-commercial, wild rose species of the Rosa genus, such as the meadow rose (R. blanda), swamp rose (R. palustris), Carolina rose (R. Carolina), prickly wild rose (R. acicularis), and burnet rose (R. spinosissima), show only minimal signs of susceptibility to RRV[4]. Currently, RRV is primarily distributed throughout the eastern United States ranging from the Eastern coasts of New England to the base of the Rocky Mountains[5]. In short, RRV is a destructive and highly lethal rose pathogen that expresses a significant threat to the commercial rose industry. This report seeks to highlight what we currently know about RRV and to highlight the areas where future research needs to be conducted.
Signs and Symptoms of RRV
Overall, RRV infection is characterized by excessive thorniness, over-zealous leaf proliferation, leaf mosaic patterning, red pigmentation, and witches' broom (see Figure 1). The symptoms of rose rosette virus (RRV) disease are quite complex and can be split up into three parts[4]. Stage 1 represents newly infected plants. Stage 1’s earliest symptoms found in susceptible Rosa spp., include a vibrant magenta almost purple coloration on the abaxial (underside) veins which extends out into leaves, reduction in flowering, and shoots have an increased density of thorns. Another common symptom found in Stage 1 is that affected leaves are deformed, chlorotic w/ red pigmentation, and elongated (see Figure 5). Stage 2 RRV infection exhibits early rosette character in which leaves display a mosaic pattern with vibrant red coloration. The leaves also tend to be highly elongated, disfigured, and exhibit a ruffled pattern. Like in Stage 1, lateral buds break dormancy early and grow before the plant is ready. The breaking from dormancy is possibly suggests that some RRV’s pathogenicity results from interference with abscisic acid signaling. Petioles are significantly shorter, which gives the classic rosette phenotype on symptomatic shoots. Growth rate on unaffected areas of the rose bush is often greatly reduced. Flowering is extremely rare in affected RRV areas of the plant. The final stage, Stage 3, is characterized by a large reduction in leaf size becoming almost hair-like and intensely red in hue. Rosette formation is full characterized forming the described witches’ broom phenotype. Petioles are short, almost all lateral buds break dormancy, growth is weak, and the whole plant is characterized by a vibrant, deep red coloration. For rose plants that are in Stage 3 most are unable to survive freezing conditions. As there is no cure for RRV, most rose plants that are susceptible to the disease will die. Typically, a mature rose plant that becomes infected with RRV, will succumb to disease after a period of three to five years[4].
Isolation History of RRV
The first indication that RRV was indeed a virus came when large virus-like particles were observed with scanning electron microscopy in Rosa multiflora and commercial roses in Northern Arkansas[6]. In the same study, the double-membrane characteristic of the spherical envelope was observed for the large virus-like particles. The next breakthrough in the isolation of RRV came with the isolation of dsRNA from infected rose tissue[7]. dsRNA, being something that is prevalent and unique to viruses, strongly suggested that the causative agent for the rose rosette disease was a virus.
Up until 1995, rose rosette disease (RRD) was thought to be caused by a virus or a phytoplasma; a phytoplasma can be equally as small as a virus[8]. A phytoplasma was ruled out as the cause of rose rosette disease, by the lack of a DAPI DNA stain in isolated cells, no reversion in symptoms when plants were treated with tetracycline, and no amplification was detected using known primers of phytoplasmas via PCR analysis [8].
The negative-sense RNA nature of RRV was finally elucidated in 2011, by using degenerate oligonucleotide primed reverse transcriptase PCR to amplify dsRNA[2]. The purification of emaraviruses from inflected plants has been challenging to researchers due to the enveloped nature of the virus particles as well as by the low titre[9]. This potentially explains why RRV and related emeraviruses were reported only having four genomic RNA segments rather than more[2][10][11]. Recently, from more sensitive analysis another three RNA segments were isolated and detected in RRV [12]. There are many things not fully understood about RRV. At the foremost of this list is the pathogenicity of RRV. Future studies need to be conducted in order to elucidate the mechanism of entry for RRV, the replication of RRV, and the possible latency of RRV.
Virion and Genome Structure
The rose rosette viron particle is of large size ranging from 120-150nm[6]. The RRV viron particle is comprised of symmetrically helical enveloped ribonucleocapsid and has been described as having a spherical shape[4][13]. Rose rosette virus is a negative-sense RNA virus and was identified in 2011 as a member of the genus, Emaravirus [2]. Like European mountain ash ringspot associated virus (EMARaV), RRV has four common RNA coding segments, RNA1-RNA4[14] as well as three other uncharacterized RNA5-7 segments[12]. The RNA1-4 segment protein products are referred to p1-p4 respectively (see Figure 4)[2]. In RRV the RNA1, RNA2, and RNA3 each contain an open reading frame (OPR) that putatively encodes for RNA-dependent RNA polymerase (RdRp, RNA1), glycoprotein precursor (RNA2), and nucleocapsid (RNA3)[15]. Fascinatingly, segmental RNA from the RRV genome was found to be uncapped, but mRNA of RRV transcripts were found to be capped with 7-methylguanylate just like all eukaryotic mRNA transcripts[2].
In RRV, RNA4 (p4) function has not be elucidated. However, RRV p4 is closely related to virus raspberry leaf blotch emaravirus p4 (RLBV, see Figure 2)[16]. In a study looking at p4 in RBLV, it was shown that the p4 protein localizes to the plasmodesmata, hinting that the protein is a viral movement protein otherly known as an MP[9]. Upon further investigation the p4 protein from RLBV rescued cell-to-cell movement of a MP-deficient potato virus X (PVX), which provides evidence that the p4 protein in RRV is likely an MP protein[9]. There are direct genetic indications that RRV p4 is a cell-to-cell movement protein with the largest piece of evidence coming from the fact that there are dnaK and ATPase motifs in the RRV RNA4 segment, which codes for p4[2]. dnaK and ATPase domains are required for plant viral movement proteins[17]. It is likely that p4 is a movement protein. However, until an experiment like that Chulang Yu and colleagues (2013) is conducted in an RRV infected host, we will not know for certain the function of RRV p4[9].
Unlike RRV, other emaraviruses such as RLBV has at least eight putatively encoding RNA segments [16]. Due to the low titre and enveloped nature of RRV, RRV may be comprised of more RNA segments[2][9]. In a follow-up isolation study by Di Bello and colleagues (2015), three new RNA genome segments were found[12]. However, it is possible fragile or low concentration RNA segment regions may have gone undetected in RRV samples. In order to build a better map of the RRV genome, future dsRNA isolation studies of RRV will have to be conducted in order to confirm that there are only seven RNA segments.
Genomic Methods of Detection for RRV:
Traditionally many viruses are detected through the use of antibody based methods. For example, enzyme linked immunosorbent assays or ELISAs are a common and effective method for quickly identifying if a particular organism is infected with say tobacco mosaic virus (TMV). Just like TMV, an ELISA can be utilized to detect the presence of RRV. A direct ELISA functions by immobilizing the target viral antigen on a polystyrene plate coated with a non-reacting protein such as BSA. After immobilization, the primary antibody is added with a conjugated enzyme. The conjugated enzyme binds specifically to the antigen as well as the primary antibody. A substrate is then added for the enzyme such as PNP solution to detect a color reaction that can quantitatively be read using a spectrophotometer. Thus, the higher the concentration of the primary antibody, the more target viral antigen is present. A few downsides with viral ELISAs are that they require a significant amount of biochemical knowledge of the virus being studied[18].It can be difficult find to monoclonal antibodies that can recognize the target virus and the antibodies themselves can be quite costly. Lastly, ELISAs are also not as fast or specific/discriminatory as other nucleic acid, PCR based methods[3][19]. Although highly specific ELISAs are; they cannot distinguish between antigenically identical analytes, i.e. different molecules recognizing the same antibody[18].
As of today, the most sensitive and effective method of RRV detection is through RT-PCR[3]. The developed RT-PCT assay by Dobhal and colleagues (2016) can detect as little as 1fg (femtogram) of RRV in roses infected with RRV (see Figure 3). The RT-PCR method outlined is a cheaper and faster analysis methodology than using standard PCR probe-based analyses[20]. The basic RT-PCR RRV identification protocol utilizes primers that were designed amplify sequences of the RRV nucleocapsid gene p3 located within the RNA3 region, which is believed to be highly conserved in RRV[2][3]. RT-PCR was optimized for RRV isolation and identification of RRV via adjustments made in annealing temperature, production of higher affinity primers, and concentrations of reagents[3].
Ecology, Epidemiology, and Transmission:
The primary vehicle in transmission of RRV is the eriophyid mite, Phyllocoptes fructiphilus[21]. As of today only eriophyid mites are known animal vector to transfer RRV (see Figure 6). Grafting has also been demonstrated to transmit RRV[2]. Eriophyid mites feed of infected RRV rose tissue and carrying virons within their probiscous, transfer RRV when the mites come into contact with another rose plant[21]. Although P. frucitphilus cannot fly, they can and do move passively long distances via air currents to nearby roses, subsequently infecting new roses[4][5]. Even though the mite RRV vectors can be transmitted through wind the risk of infection for roses that 150-300m away from another infected rose plant is considered minimal[4]. The fact that the close proximity of infected host rose plants to unaffected host rose plants is needed for transmission (~20m); is immensely valuable to landscapers and rose horticulturalists[4]. Transmission of RRV can subsequently be mitigated simply by placing a large distances between rose planting.
RRV was first detected in Manitoba Canada, California, and Wyoming[1]. Since then the disease has spread across the United States and by the early 2000s cases of RRV had been detected in all of the 48 states with the greatest impact being felt in East of the Rocky Mountains[22]. The host abundance is larger/more widespread in the Eastern U.S. as well as the fact that P. fructiphilius is less fit for the drier climates of Western States[22]. It has been postulated that the spread of RRV has been expedited by the widespread coverage of the invasive species Rosa multiflora (Multiflora-Rose)[23]. R. multiflora is native to Eastern China, Korea, and Japan that is highly susceptible to RRV[23]. R. multiflora became a ubiquitous invasive species across the Midwestern and Eastern United States starting after the 1930s. Starting in the 1930s, the U.S. Soil Conservation Service recommended and distributed R. multiflora seeds and saplings for use in erosion control and to be used as “living fences” for livestock. However, due to the proliferative nature of R. multiflora, the plant soon became a common pest/noxious weed in several U.S. states. Because of how widespread R. multiflora is across the U.S., it serves as RRV pool upon P. fructiphilus mites can transmit the disease to commerically relevant species of Rosa.
In a field experiment, RRV was demonstrated to increase R. multiflora mortality from 1.1 to 44.3%, for 180 plants across a two year period[24]. Since rose rosette disease effectively infects via P. fructiphilus and kills the invasive plant R. multiflora, RRV has been proposed as a potential biological control for R. multiflora[23]. The downside of having a significant RRV pool is that the disease can be more readily transferred to commercially relevant roses.
Evolution
RRV’s exact aetiology is currently unknown. Many conjecture that RRV came from the indigenous Rosa multiflora populations in China, Korea, and Japan (Doudrick et al., 1986). However, there has not been evidence presented supporting this claim. One thing that we do have a clearer picture of is RRV’s phylogenetic history. An isolation and phylogenetic study of RRV conducted by Laney et al. (2011), classified RRV as an Emaravirus with close connections to European mountain ash ringspot-associated virus (EMARaV) and Fig mosaic virus (FMV). From isolated p3 and p4 protein domains in RRV, they demonstrated 97%-99% exact nucleotide identities to FMV and EMARaV p3 and p4 protein domains (Laney et al., 2011). Based on RNA-dependent RNA polymerase sequence similiarity, Emaraviruses are distantly related to other negative-sense RNA viruses, such as tospoviruses and tenuivirus (Benthack et al., 2005).
A fascinating aspect of RRV is the four part RNA, segmented genome. Evolutionarily, an RNA segmented genome could theoretically allow for replication to be spread out of more of the host cell’s resources, thus making replication faster for RNA segmented genomes (Chao, 1991). Ultimately, this would confer a replicative advantage for the RNA segmented genome. Nevertheless, this has only been theoretically conducted and not demonstrated in a viral model setting (Chao, 1991). Another potential advantage of having a segmented genome is that the rate of reassortment would be predicted to be higher because there are simply more parts to be linked together or shuffled around. Reassortment in segmented RNA genomes events could potentially remove deleterious configurations and/or generate advantageous genomic configurations (Chen and Holmes, 2008).
The hypothesis that segmented genomes have a recombination advantage over non-segmented genomes has been postulated. The rationale is that the segments will potentially be closer together allowing for homologous regions to interact and recombine more readily in segmented RNA genomes. However, at this time, the data does not support that recombination occurs more readily in segmented RNA genomes (Fraile et al., 1997; Chare et al., 2003). In an experiment looking at the tripartite RNA cucumber mosaic cucumovirus, recombination and reassortment rates were quantitatively observed to be infrequent. Only a 7% recombination rate was observed between segments and the recombinants were conserved within the population (Fraile et al., 1997).
A problem with long single stranded RNA (ssRNA) viruses is their instability as chain length grows (Ojosnegros et al., 2011). Short chains are much more stable than long ssRNA chains. For the sake of stability, less coding genetic material can be add to the RNA chain, which in turn means less coding information can be fit on the RNA chain. Thus, there is a trade-off between chain length (the total amount of genetic material) and particle stability. The segmentation of the RNA genome presents itself as a possible solution. Data has been presented that suggests virally segmented genomes serve as a molecular solution to the trade-off between the stability of the RNA segment and length of the RNA segment (Ojosnegros et al., 2011). Genomic segmentation serves as a way to maximize the genetic content of a genome while overcoming the detrimental effect of RNA chain length instability.
Commercial Impact
Roses (Rosa spp.) are of immense commercial importance for their use in landscaping, aesthetic value, industrial products, and cosmetic products. To emphasize the economic importance of roses; roses have a total wholesale value of $194 million in the United States (Babu, 2014).
Although, there has not been an official report on the economic impact of RRV related damages, it is believed to be well into the millions. The disease is primarily contained to the United States but recently RRV has been spotted in India (Chakraborty et al., 2017). The spread of RRV outside of North America has been raising concerns throughout the globe, considering that two-thirds of all of the $40billion dollar cut flower market is comprised of roses. RRV, although difficult to transmit, has a high lethality and can decimate densely populated rose populations. For example, in 2012, RRV decimated the Tulsa Municipal Rose Garden in which thousands of rose plants had to be eradicated (Stanley, 2013). Due to widespread nature of RRV across the southern U.S. and Midwest it is proving to be a common pest for the average home gardener and nurseries that carry rose plants.
Management
The most effective management of RRV is through management the vector of transmission, P. fructiphilus. Listed below are effective and common management practices that can greatly curtail of the transmission and severity of RRD from RRV (Hoy, 2013).
1.Once a rose has been identified with RRV, the plant should be removed and either placed in a plastic air-tight bag for disposal or be burned.Removal of infected RRV roots should also be conducted.
2.Pruning roses can potentially eliminate mites and eggs. The eggs and mites are predominately found within the new growth areas and petiole areas of the rose.
3. Removal of R. mulitflora roses in the area of your cultivar roses, which is best done by mechanical removal, i.e. cutting them down during the growing season. Herbicide applications can also be made to stem of muliflora roses to hasten their death.
4. Currently it is unknown which pesticides are effect control measures for the reduction of eriophyid mites as they often hidden from observation due to their placement in the buds on growing apical tissue.
5. Phytocides, although unproven, may be an effective measure in managing the population of the population of eriophyid mites.
Solely chemical control of eriophyid mites is not advised due to the fact that at least nine species of the mites have developed significant pesticide resistance (Hoy, 2013). Instead, a combination of the methods listed above is the most effective way to stem the tide of transmission of RRV.
Currently, research is being conducted on making RRV resistant roses (Dobhal et al., 2016). There are two main approaches that are being done to create an RRV resistant rose. The first involves traditional breeding between commercially susceptible rose varieties and resistant non-susceptible wild Rosa varieties. The alternative approach is through genetic engineering in which researchers are trying to identify resistance genes or R-genes that confer resistance to RRV (Hoy, 2013). One potential way in which researchers can possible discover and isolate R-genes that confer RRV resistance is through Quantitative Trait Locus (QTL) mapping of nucleotide-binding site/leucine-rich repeat like sequences (NBS-LRRs) (Meyers et al., 2003). NBS-LRRs are genetic motifs commonly found in R-genes (Meyers et al., 2003).
Conclusion
Rose rosette virus is a serious plant pathogen that has a high rate of mortality for an infected plant. Ecologically, RRV is serving as a biological control for the invasive species R. multiflora but conversely is a harmful disease to ornamental rose species. Research into breeding/genetically engineering resistant RRV roses should be conducted.
References
- ↑ 1.0 1.1 Conners, I.L. Twentieth Annual Report of the Canadian Plant Disease Survey 1940; Department of Agriculture: Ottawa, Canada, 1941; p. 98.
- ↑ 2.0 2.1 2.2 2.3 2.4 2.5 2.6 2.7 2.8 2.9 Laney A., Keller K., Martin R.,& Tzanetakis I. A discovery 70 years in the making: characterization of the Rose rosette virus. 01 July 2011, Journal of General Virology 92: 1727-, doi: 10.1099/vir.0.031146-0
- ↑ 3.0 3.1 3.2 3.3 3.4 Dobhal, S., Olson, J. D., Arif, M., Suarez, J. A. G., & Ochoa-Corona, F. M. (2016). A simplified strategy for sensitive detection of Rose rosette virus compatible with three RT-PCR chemistries. Journal of virological methods, 232, 47-56. doi:10.1016/j.jviromet.2016.01.013
- ↑ 4.0 4.1 4.2 4.3 4.4 4.5 4.6 4.7 Epstein, A.H., & Hill, J.H. (1999). Status of rose rosette disease as a biological control for multiflora rose. Plant disease, 83(2), 92-101. doi:10.1094/PDIS.1999.83.2.92
- ↑ 5.0 5.1 Windham, M., Windham, A., Hale, F., & Amrine Jr, J. (2014). Observations on Rose rosette disease.
- ↑ 6.0 6.1 Gergerich, R.C., & Kim, K.S. (1983). A description of the causal agent of rose rosette disease. Arkansas Farm Research, 32(3)
- ↑ R., Hill, J.H., & Epstein, A.H. (1990). Double-stranded RNA associated with the rose rosette disease of multiflora rose. Plant disease, 74(1), 56-58
- ↑ 8.0 8.1 Epstein, A.H., & Hill, J.H. (1995). The Biology of Rose Rosette Disease: A Mite‐associated Disease of Uncertain Aetiology. Journal of Phytopathology, 143(6), 353-360. doi:10.1111/j.1439-0434.1995.tb00275.x
- ↑ 9.0 9.1 9.2 9.3 9.4 Yu, C., Karlin, D.G., Lu, Y., Wright, K., Chen, J., & MacFarlane, S. (2013). Experimental and bioinformatic evidence that raspberry leaf blotch emaravirus P4 is a movement protein of the 30K superfamily. Journal of General Virology, 94(9), 2117-2128.doi:10.1099/vir.0.053256-0
- ↑ Mielke, N., & Muehlbach, H. P. (2007). A novel, multipartite, negative-strand RNA virus is associated with the ringspot disease of European mountain ash (Sorbus aucuparia L.). Journal of General Virology, 88(4), 1337-1346.doi: 10.1099/vir.0.82715-0
- ↑ Elbeaino, T., Digiaro, M., Alabdullah, A., De Stradis, A., Minafra, A., Mielke, N., Castellano, M.A., & Martelli, G. P. (2009). A multipartite single-stranded negative-sense RNA virus is the putative agent of fig mosaic disease. Journal of General Virology, 90(5), 1281-1288.doi: 10.1099/vir.0.008649-0
- ↑ 12.0 12.1 12.2 Di Bello, P. L., Ho, T., & Tzanetakis, I. E. (2015). The evolution of emaraviruses is becoming more complex: seven segments identified in the causal agent of Rose rosette disease. Virus research, 210, 241-244.doi:10.1016/j.virusres.2015.08.009
- ↑ Kim, K. S., Ahn, K. K., & Gergerich, R. C. (1995). Double membrane-bound virus-like particles associated with rose rosette and some other diseases transmitted by eriophyid mites. In Proc Int Symp Rose Rosette. Iowa State University Press, Ames (pp. 39-41).
- ↑ Ishikawa, K., Maejima, K., Komatsu, K., Netsu, O., Keima, T., Shiraishi, T., Okano, Y., Hashimoto, M., Yamaji Y., & Namba, S. (2013). Fig mosaic emaravirus p4 protein is involved in cell-to-cell movement. Journal of General Virology, 94(3), 682-686.doi:10.1099/vir.0.047860-0
- ↑ Mielke-Ehret, N., & Mühlbach, H.P. (2012). Emaravirus: a novel genus of multipartite, negative strand RNA plant viruses. Viruses, 4(9), 1515-1536.doi:10.3390/v4091515
- ↑ 16.0 16.1 McGavin, W. J., Mitchell, C., Cock, P. J., Wright, K. M., & MacFarlane, S. A. (2012). Raspberry leaf blotch virus, a putative new member of the genus Emaravirus, encodes a novel genomic RNA. Journal of General Virology, 93(2), 430-437.doi:10.1099/vir.0.037937-0
- ↑ Alzhanova, D. V., Napuli, A. J., Creamer, R., & Dolja, V. V. (2001). Cell‐to‐cell movement and assembly of a plant closterovirus: roles for the capsid proteins and Hsp70 homolog. The EMBO journal, 20(24), 6997-7007.doi:10.1093/emboj/20.24.6997
- ↑ 18.0 18.1 Baker, G., Dunn, S., & Holt, A. (2007). Handbook of Neurochemistry and Molecular Neurobiology: Practical Neurochemistry Methods (Vol. 6). Springer Science & Business Media.
- ↑ Dai, J., Cheng, J., Huang, T., Zheng, X., & Wu, Y. (2012). A multiplex reverse transcription PCR assay for simultaneous detection of five tobacco viruses in tobacco plants. Journal of virological methods, 183(1), 57-62.doi:10.1016/j.jviromet.2012.03.029
- ↑ VanGuilder, H. D., Vrana, K. E., & Freeman, W. M. (2008). Twenty-five years of quantitative PCR for gene expression analysis. Biotechniques, 44(5), 619.doi:10.2144/000112776
- ↑ 21.0 21.1 Amrine, J. W., & Hindal, D. F. (1988). Rose rosette: a fatal disease of multiflora rose (Vol. 147). West Virginia University, Agricultural and Forestry Experiment Station.
- ↑ 22.0 22.1 Amrine Jr, J. W. (2002). Multiflora rose. Biological control of invasive plants in the eastern United States, 265-292.
- ↑ 23.0 23.1 23.2 Amrine, J. W. (1996). 4.1. 2 Phyllocoptes fructiphilus and biological control of multiflora rose. World Crop Pests, 6, 741-749.doi:10.1016/S1572-4379(96)80050-9
- ↑ Amrine Jr, J. W., Hindal, D. F., Williams, R., Appel, J., Stasny, T., & Kassar, A. (1990). Rose rosette as a biocontrol of multiflora rose, 1987-1989. In Proceedings of the 43rd Annual Meeting of the Southern Weed Science Society. (pp. 316-320).
Authored for BIOL 238 Microbiology, taught by Joan Slonczewski, 2017, Kenyon College.