HIV Drug Resistance
Introduction
By Lillian Spetrino
History of HIV
In 1981, five previously healthy Los Angeles men presented with pneumocystis pneumonia, caused by a fungus that results in the lungs filling with fluid and becoming badly scarred exclusively in people with weakened immune systems. After much dedicated research, it was determined that the cause of these weakened immune systems was a lentivirus termed “HIV” for Human Immunodeficiency Virus. Important research has been conducted to develop drugs that can slow the rise of HIV in infected people, so that onset of AIDs (Acquired Immune Deficiency Syndrome) is delayed (Zimmer).
HIV Structure and Life Cycle
There are two known types of HIV – HIV type 1 and HIV type 2. HIV-2 is less pathogenic than HIV-1 and is thus less widely spread. HIV-1 is a spherical enveloped virus about 120 nm in diameter composed of a protein complex that controls membrane fusion with host CD4+ cells (Chan). The genome of HIV is comprised of two copies of (+) single stranded RNA. HIV infects a variety of immune cells including CD4+ T cells, macrophages, and microglial cells. The transmission of bodily fluids including blood, semen, vaginal fluid, pre-ejaculate, or breast milk transmits infection. Sexual intercourse is the foremost manner of transmission, but is also common through intravenous drug users.
HIV enters the CD4 T cell though tight binding to membrane proteins, causing a conformational change that fuses the membranes and allows entry of the viral capsid in the host cell. Once inside the cell, HIV RNA and reverse transcriptase, integrase, ribonuclease, and protease are used to reverse transcribe the (+) RNA into cDNA, which is transported to the nucleus and into the host cell genome catalyzed by integrase. The virus is then transcribed into mRNA and spliced, exported into the cytoplasm, translated and packaged into virions. These immature virions are transported to the plasma membrane where protease cleaves the gag polyproteins into the matrix, capsid, and nucleocapsid.
HIV Therapy
Prior to 1996, when combination antiretroviral therapy was developed HIV was considered a fatal illness. However, with this development, HIV is now considered a manageable, albeit chronic, disease (Bangsberg). Antiretroviral therapy has decreased HIV-associated morbidity and mortality and changed the disease into a chronic, but controllable illness (Panel on Antiretroviral Guidelines for Adults and Adolescents), as opposed to a death sentence. Antiretroviral therapy is highly effective in transmission prevention between sexual partners (Panel on Antiretroviral Guidelines for Adults and Adolescents). The treatment goals of antiretroviral therapy are to reduce HIV-associated illness and prolong the length and quality of life, repair and preserve immunologic function, suppress plasma HIV viral load, and block HIV transmission (Panel on Antiretroviral Guidelines for Adults and Adolescents). However, an undesired result of antiretroviral therapy can be mutations that lead to decreased drug vulnerability (Novak). Drug-resistance HIV patients have restricted treatment options and have been found to have reduced response to therapy (Novak).
There are four major classes of HIV treatment drugs. One such class is nucleoside and nucleotide analogues. This drug terminates the DNA chain and inhibits reverse transcription of the viral RNA. Another drug class is nonnucleoside reverse-transcriptase inhibitors, which bind and inhibit reverse transcriptase. Additionally, entry inhibitors block the entry of HIV virions into their target cells. Lastly, protease inhibitors target the viral enzyme protease. Protease cleaves the precursor proteins gag and gag-pol, which is important for viral maturation (Clavel). Multiple drug resistance requires ongoing rounds of viral replication in order to create mutations that confer resistance.
Combination therapy is the customary treatment for patients infected with HIV-1. This is the use of three drugs from at least two classes (Panel on Antiretroviral Guidelines for Adults and Adolescents) in order to increase the barrier to resistance. Combination therapy helps to decrease the likelihood of resistance because it reduces HIV replication and thus reduces the likelihood that a resistance mutation will occur. Since resistance requires multiple mutations to develop against all the drugs in the treatment, combination therapy can defend against resistance. However, combination therapy is unable to wholly eliminate resistance to antiretroviral drugs because highly active antiretroviral therapy (HAART) cannot completely overpower viral replication (Smyth).
The goal of HIV treatment is to reduce the amount of virus to indiscernible levels, or less than 50 copies of the virus per mL, ideally 24 weeks post combination therapy. If viral load is found to be higher than 200 copies per mL, further testing must be considered to determine the cause of the virologic failure, which could potentially be resistance. Another way to test how well the treatment is working is to use CD4 cell counts. If the number of CD4 cells is less than 50-100 cells per mL after a year (with 25% fluxuation), it may be indicative of insufficient antiretroviral therapy effectiveness. If there are low viral counts accompanied with low CD4 cell counts, this is termed “immunologic nonresponse” or “immunologic failure” (Panel on Antiretroviral Guidelines for Adults and Adolescents and Gazzola)
Resistance
HIV has a high rate of evolution (Smyth) and therefore abnormally elevated genetic diversity. This genetic variability is a result of the rapid replication cycle. The replication cycle of HIV has a generation time of about 2.5 days, producing between 1010 and 1012 new virions each day. This in combination with the high mutation rate (approximately 0.2 errors by reverse transcriptase per genome during each replication cycle) and the ability to frequently recombine (Rambaut 2004), confers a high mutation rate. High mutation rates can cause drug resistance because modulation of HIV-1 mutation rate by reverse transcriptase can cause polymorphisms that alter replication fidelities resulting in drug resistance (Smyth). Recombination is associated with resistance because drug resistance mutations have been shown to alter the frequency of template switching (Smyth). Template switching is when there is a base-pairing interaction between the 3’ end of a strong-stop DNA and a complementary RNA sequence (Luo) resulting in the production of a recombinant provirus (Bonhoeffer). The genetic diversity in the form of a viral quasispecies is associated with the failure of antiretroviral therapies due to drug resistance (Smyth). Because of the nature of HIV-1 replication and the tendency for rapid selection, high-level drug resistance is a potential hurdle to overcome when treating HIV (Smyth).
Another potential cause of drug resistance in HIV is incomplete adherence. According to the World Health Organization, adherence to long term therapy is “the extent to which a person’s behavior – taking medication, following a diet, and/or executing lifestyle changes, corresponds with agreed recommendations from a health care provider” (World Health Organization). Incomplete adherence therefore is failure to follow such instructions from a health care professional and has often been thought to lead to drug resistance because at low adherence, there is not as much selective pressure to force mutations to arise that may trigger drug resistance. However, recent data has suggested that each regiment class might have a special relationship between adherence and resistance (Bangsberg).
HIV-1 Protease
Structure and Function
HIV-1 protease is a homodimer composed of two identical subunits of 99 amino acids. Great plasticity and polymorphisms have been observed in 1/3 of the amino acids (Wensing). Also included are two molecular “flaps” that move up to 7 Å when the enzyme is charged with a substrate (Miller). This movement of the flaps is what allows the substrate to enter or depart the cleft (Marseveen). The active site is located between identical subunits and has the classic Asp-Thr-Gly sequence found in many aspartic proteases. More specifically, the catalytic activity of the enzyme is found between the Asp25 residues.
Mature infectious virus particles are generated when HIV protease cleaves viral Gag and GagPol precursor proteins (Wensing). Gag encodes capsid, nucleocapsid, and matrix proteins (Slonczewski), while GagPol encodes RT, integrase, and protease (Slonczewski). Mechanistically, water acts as the nucleophile in combination with aspartic acid to hydrolyze the peptide bond (Jaskolski). This cleavage occurs during or shortly after the assembled viral particles are expelled from host cells and are therefore only essential for viral maturation, but are not required for viral production (Wensing).
Protease Inhibitors
Protease inhibitors utilize competitive regulation in order to inhibit HIV-1 protease. The use of the drug results in production of non-infectious virions because inhibition of protease yields immature virions due to lack of cleavage of Gag and GagPol. Protease inhibitors bind viral protease as the natural substrate would, but occupy more space than natural substrates (Wensing), ideally resulting in reduction of mature virions.
Preferred protease inhibitor-based regimens include Ritonavir-Boosted Atazanavir and Ritonavir-Boosted Darunavir (Panel on Antiretroviral Guidelines for Adults and Adolescents). Other protease inhibitors approved for clinical use are saquinavir, ritonavir, indinavir, nelfinavir, amprenavir, lopinavir, atazanavir, tipranavir and darunavir. Most protease inhibitors utilize competitive inhibition and include a hydroxyethylene center that inhibits cleavage of the protein precursors.
One issue with protease inhibitors is that many of them have poor oral bioavailability or short half lives, which causes patients to require large amounts of the drug more frequently. This leads to a potential problem with adherence which can lead to resistance. In addition, large volumes of the protease inhibitor can have short term toxic effects, such as gastrointestinal side effects, rash, renal colic, dry skin, and paronychia. Most concerning for patients was the resulting fat redistribution linked with protease inhibitors in 2-83% of patients on protease inhibitor therapy, further increasing the likelihood of inadequate adherence (Walmsley).
In order to combat these issues with protease inhibitors, there was a move towards using nonnucleoside reverse transcriptase inhibitors. However this came with its own set of issues: pregnant women cannot use nonnucleoside reverse transcriptase inhibitors because they can cause birth defects and they can cause hepatotoxicity in women with CD4 counts less than or equal to 250 cells per mm3 and men with CD4 counts less than or equal to 400 cells per mm3. Therefore, the lower pill burden was incapable of overcoming the negative side effects of nonnucleoside reverse transcriptase inhibitors, especially when the potential to become resistant to both nonnucleoside reverse transcriptase inhibitors and nucleoside reverse transcriptase inhibitors is possible (Walmsley). Therefore, improved protease inhibitors with more accessible dosing schedules was required. The solution scientists came up with was to “boost” the protease inhibitors.
Protease inhibitors are metabolized in the liver by CYP3A isoenzymes. Therefore if cytochrome p450 is induced or inhibited, metabolism of protease inhibitors may be impacted (Panel on Antiretroviral Guidelines for Adults and Adolescents). This is the reason why many protease inhibitors are often boosted with ritonavir, a CYP inhibitor. Boosted protease inhibitors significantly increase the levels of protease inhibitors in the blood plasma. However, this pharmacologic boosting can potentially raise protease inhibitor levels too much, causing deleterious effects, particularly gastrointestinal and hypertriglyceridemia (Walmsley).
There are some advantages and disadvantages to using protease inhibitors in HIV treatment. They are advantageous in the sense that it is more difficult to genetically develop resistance than it is for other HIV treatment drugs such as non-nucleoside reverse transcriptase inhibitors. Another advantage is that at the time of treatment failure, protease inhibitor resistance is uncommon when the protease inhibitor is ritonavir-boosted (Panel on Antiretroviral Guidelines for Adults and Adolescents). Unfortunately, there are also disadvantages to using protease inhibitors. Some metabolic complications that can arise are dyslipidemia, insulin resistance, and hepatotoxicity as well as gastrointestinal adverse effects. Also, there is a potential drug interaction is with CYP3A4 inhibitors and substrates, this interaction being more pronounced with ritonavir-based regiments (Walmsley).
Protease Inhibitor Resistance
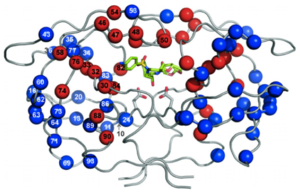
Viral reverse transcriptase lacks proofreading activity, and thus led to viral variants which retain mutations (Maarseveen). As the number of protease inhibitors has increased, it is important to be able to delineate how each protease inhibitor mutation impacts resistance to other protease inhibitors (Rhee). Estimated prevalence for protease inhibitor resistance is 0.7% (Novak).
Protease inhibitor mutations on drug susceptibility and replicative capacity
High level resistance requires several mutations, and these mutations must reduce the ability of the virus to replicate. Boosted protease inhibitor regimens have been found to be more effective at decreasing viral replication than unboosted protease inhibitor regimens. In addition, it was discovered that protease mutations that have the largest influence on protease inhibitor vulnerability are those that are not polymorphic in the absence of protease inhibitor therapy (Rhee).
Resistance Mechanism
Protease inhibitor resistance usually begins with mutations likely to reduce binding affinity with the target, typically in the active site, thereby reducing replication capacity (Bangsberg). It is considered to be a stepwise process (Wensing) whereby an alteration in the active site of the protease is altered in some way. Typically, there is a substitution in the substrate-binding cleft of the viral protease resulting in enlargement of the catalytic site. This enlargement leads to decreased binding to the inhibitor (and therefore causing a decrease in drug sensitivity) as well as the natural substrate, which leads to decreased viral replication. These can be considered primary, or major, mutations. Secondary, or minor, mutations may also occur, however these do not typically have significant effect on resistance phenotype alone. Nonetheless, secondary mutations in conjunction with major mutations will improve replication of viruses.
Another potential mechanism of resistance is through an amino acid insertion. Amino acid insertions ranging from 1 to 6 amino acids have been observed during protease inhibitor-based antiretroviral therapy (Wensing). Such insertions may lead to decrease in protease inhibitor susceptibility and slightly improve viral replication, but only contribute to resistance to protease inhibitors when in combination with other mutations in protease or Gag protein. Insertions have a positive correlation with protease mutations associated with protease inhibitor resistance whose usage has increased in recent years (atazanavir, lopinavir, amprenavir, and tipranavir) (Wensing)
Other mechanisms of resistance include mutations in the Gag protein in or near the protease cleavage sites. This is typically in response to the altered substrate-binding cleft of the mutant drug-resistant viral protease (Wensing). Mutations in viral protease in combination with mutations in Gag protein increase protease inhibitor resistance (Wensing). Another alternative pathway is a substitution in the Gag NC/p1 cleave site without any alterations in the viral PR sequence that causes protease inhibitor resistance leading to increased gag processing (Wensing). Furthermore, cleavage site (CS) mutations have been found to accumulate in protease inhibitor-resistant HIV isolates, correlating with the presences of three or more protease inhibitor resistance mutations. Mon-cleavage site mutations in Gag were also found in the frame-shift regulating site were also observed to be associated with resistance. In one experiment by Knops, 25% of HIV isolates from patients failing protease inhibitor treatment without protease mutations possessed Gag mutations associated with protease inhibitor resistance (Knops). These Gag mutations could potentially indicate the evolution of protease inhibitor resistance, even without mutations in the protease enzyme.
Specific Drug Resistance
Nelfinavir
Nelfinavir is a protease inhibitor that binds tightly to protease in the active site. The drug cannot be boosted, meaning it cannot be paired with ritonavir to pharmacologically increase amounts of nelfinavir in the blood by inhibiting metabolism of nelfinavir by cytochrome p450 (Walmsey). Because of this, nelfinavir is less successful at reducing viral load than protease inhibitors that can be boosted.
The protease mutation D30N is the mutation associated with resistance in nelfinavir treated patients. However, it only confers moderate levels of phenotypic resistance, suggesting that in order to develop resistance to nelfinavir, the patient must be exposed to high levels of the drug. This is in opposition to the non-adherence theory of resistance. In Bangsberg’s experiment, clinically derived patient samples were treated in vitro at variable concentrations of nelfinavir in order to simulate different levels of adherence. It was observed that resistance mutations were only observed after relatively high levels of drug exposure. In fact, 85% to 95% adherence was found to be the optimum adherence range for the selection of nelfinavir resistance (Bangsberg).
Lopinavir (Bangsberg)
Lopinavir is a protease inhibitor that is ritonavir-boosted. Drug resistance is uncommon in this case when patients are treatment-naïve, most likely because more than one mutation is required to generate resistance. These mutations must reduce the reproductive capabilities of the virus. Administration of ritonavir-boosted lopinavir increases’ the amount of lopinavir in the bloodstream, which makes it hard for the virus to be replicated, even when the patient is non-adherent. This is likely the case for other boosted protease inhibitor combinations.
Pharmacogenomic variation
Differing polymorphisms in CYP3A and CYP2C genes may lead to different adherence-resistance relationships, which may influence drug resistance.
Resistance Management
The first signs of drug resistance is an increase in plasma viremia (“a marker of antiviral activity and real-time phenotypic evaluation of viral isolates for the emergence of resistance” [Davey]) above the normal limits. Once it is determined that the increased levels are due to resistance, choosing therapy for treatment is a nuanced interaction between the patient and the heath care professional. Typically, an almost monotherapy regimen is developed using newly developed agents that have novel viral targets. (Cortez)
One option discussed by Dr. Scholmo Staszewski, from the University of Frankfurt in Germany, is the role of “double PI” boosting as a potential therapy for patients with drug resistance, but remains skeptical and suggests further studies into drug combinations before use clinically.