Rhodopseudomonas palustris and extracellular electron transfer
Introduction
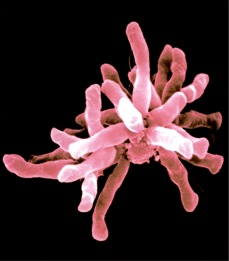
All life forms need energy to grow and survive. In order to grow, an organism needs energy to generate new cells from inorganic substances. For most organisms, this energy is derived from basic reduction-oxidation chemistry. Redox chemistry involves the transfer of electrons between two species, and this simple exchange is fundamental to processes like cellular respiration and photosynthesis. Photosynthesis occurs when carbon dioxide is reduced into sugars and water is oxidized to oxygen. Respiration, on the other hand, oxidizes sugars to yield carbon dioxide and water. Aside from these most basic processes, microbes are capable of yielding energy from virtually any chemical reaction that our biosphere allows. Rhodopseudomonas palustris is known by microbiologists as one of the most metabolically versatile bacterium on earth. R. palustris is a rod-shaped, gram-negative bacterium belonging to the proteobacteria family. It is typically found in soil, marine coast sediments and pond water. R. palustris have cells that are motile, exhibit polar growth, and reproduce via budding (Figure 1).
Metabolism
R. palustris fall under the category of purple non-sulfur bacteria, which are typically photohetorophic. Yet R. palustris is unique in its ability to switch between photoheterotrophy, photoautotrophy, chemoautotrophy, and chemoheterotrophy (Figure 2). Environmental conditions, such as the availability of light, determine which class of metabolism the bacterium uses. When light is abundant, electrons enter the respiratory chain of donors and acceptors from a photo-excited chlorophyll, bacteriochlorophyl b. In addition to bacteriochlorophyl, R. palustris have developed intracytoplasmic membranes called thylakoids that help catalyze photosynthetic reactions under anaerobic conditions.
In the presence of oxygen, the bacterium can degrade and accept electrons from performed organic molecules such as sugars and benzoates. R. palustris is particularly adept at degrading sturdy, aromatic compounds and reducing them to usable substrates, eventually forming acetyl CoA and carbon dioxide. The acetyl CoA can then enter the triboxylic acid cycle (TCA) to produce energy. This bacterium has been of great use in removing environmental pollutants, which typically contain benzene. Additionally, R. palustris can also utilize lithotrophy to acquire energy. This means it can accept electrons from inorganic compounds such as reduced sulfur and more prevalently, ferrous iron Fe(II) and channel them through the ETS to generate ATP.
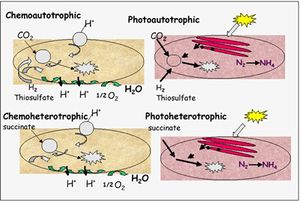
Extracellular electron transfer
Microbes typically require their electron donors and acceptors to be in solution. This way, the molecules donating or accepting the electrons can enter the cell of the microbe and be more readily available. Remarkably, a phylogenetically diverse branch of anaerobically respiring microbes can take electrons from external materials in the solid-phase. This process is known as extracellular electron transfer (EET). Little is known about EET and it is of recent interest to better understand microbe-mediated processes such as geochemical cycling, power generation in electrochemical cells, and bioremediation. R. palustris has been identified as a bacterium capable of EET, and a team led by Peter Girguis at Harvard recently examined R. palustris and its versatile metabolism to pinpoint the genetic determinants of EET and the molecular mechanisms that underlie it.
Bioelectrochemical systems to stimulate electron transfer:
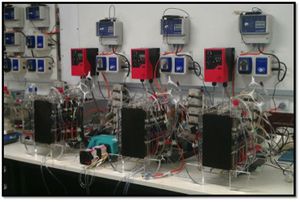
In this experiment conducted in July of 2013, Girguis et. al submerged electrodes in a bioreactor and allowed them to come into contact with R. palustris cells. The role of these bioelectrochemical systems (Figure 3) was to generate a current that mimicked the reduction potential of extracellular solid-phase minerals that R. palustris could use to metabolize. The researchers set up three different bioelectrochemical systems with differing conditions: In the first treatment, the bioreactor containing an electrode passing current was illuminated. In the second treatment or the “dark treatment,” the bioreactor was non-illuminated. In the third treatment also known as the control, illuminated bioreactors contained electrodes that had open circuits and thus passed no current.
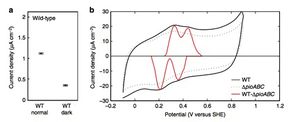
The researchers found that the bacterium exhibited the highest rate of electron uptake, measured in its current density, in illuminated treatments (Figure 4). Although significantly lower, current uptake was detected in the dark treatments. This puzzled the researchers because they had previously known that expression of ruBisCO, a key enzyme involved in carbox fixation, and the microbes ability to take up electrons are stimulated by light. They attributed this quandary to the fact that when light is unavailable, ATP cannot be synthesized through photosynthesis, yet cellular metabolism can continue as long as an electron donor is present. When light is available, however, R. palustrisis able to use photic energy to synthesize ATP from the electron transfer system without an electron donor. Electron donors are necessary only to produce reducing equivalents such as NADPH for cellular metabolism. Thus, the researchers believed that the observed current uptake in the dark treatment was simply showing the exchange of electrons from the electron donor. As expected, the electron uptake by R. palustris in the dark treatment was significantly lower than uptake in the light treatment due to the fact the ATP generated in the light treatment is used for more energy-intensive cellular processes and therefore requires a higher level of electron uptake.
Genetic mechanisms involved in EET
The PioABC operon:
These findings were insightful because it revealed that R. palustris was capable of taking up electrons from solid-phase materials if it was able to take up electrons from current generated by an electrode. Ferrous iron was not necessary for the bacterium as researchers previously thought. To examine which genetic component was at work here, the researchers turned to the gene sequence that the bacterium uses when it obtain electrons from Fe(II). They knew that the proteins involved in the electron transfer from Fe(II) to the electron transport system are coded by the pioABC operon. The pioABC system, it turns out, also affects the electron uptake that was observed from the electrode. By inoculating strains of R. palustris to render them pioABC deficient (ΔpioABC) and then comparing these strains with the wild-type strains, they found that the mutant accepted 30% less current from the electrode than the wild-type strain in the light treatments. In addition, expression of the pioABC proteins was upregulated in the wild-type strain during electron uptake. The operon in the inoculum strain, however, exhibited much lower levels of upregulation. Together, this data suggests that the pioABC operon is one of the key molecular mechanisms responsible for extracellular electron transfer within R. palustris.
Another interesting finding was that certain genes were upregulated during electron uptake depending on if the bacterium formed biofilms or remained as a planktonic cell. When the wild-type strain formed biofilms in the light treatment, expopolysaccharide (EPS) genes were upregulated. These genes were also upregulated in biofilms of the ΔpioABC strain in the light treatment. In wild-type planktonic cells, which refers to cells that did not form biofilms on the electrode, a homologue gene to the pioC was upregulated during electron uptake. Further work needs to be done to distinguish how this specific encoded protein increases planktonic cell electron uptake and how EPS genes aid in the uptake of electrons for biofilms of this bacterium.
Expression of ruBisCO:
The researches utilized transcriptome analysis to sequence RNA strains of R. palustris to examine the role of the enzyme ruBisCO in electron uptake. RuBisCO is essential to all photosynthetic organisms because it is responsible for the conversion of carbon dioxide to energy-rich molecules like glucose. It does this by catalyzing the carboxylation of ribulose-1,5-bisphosphate, yielding two molecules of 3-phosphoglycerate which can then be reduced by NADPH in the Calvin cycle. R. palustris is able to express two different forms of ruBisCO, noted as Form I and II. Both forms perform similar functions and are responsible for the fixation of carbon dioxide, yet Girguis et al. found that form I was more highly expressed in the wild-type strain in the light treatments than form II. Also, expression of ruBisCO form I was significantly lower in the dark treatments. It has been known that in the presence of light, ATP and NADPH are produced, and these energy carriers have been shown to positively regulate ruBisCO expression. In the dark, these energy-rich metabolites are much less abundant and therefore ruBisCO expression decreases.
R. palustris purposefully remains towards the surface of soil and water in order to remain close to sunlight. This way, ruBisCO can be activated more regularly and electron uptake can occur. Yet the researches found that much of the ferrous minerals that it acquires electrons from lie deep within sediment because they are insoluble. In this way, R. palustris is unique among bacteria in its ability to access energy from both underground minerals and sunlight.
Nitrogen fixation and Hydrogen Production
Another important aspect of R. palustris's metabolism is its highly efficient way of producing hydrogen. Hydrogen is a particularly efficient fuel source because it can be easily combined with oxygen to produce electricity, heat, and water. Many issues arise with using hydrogen as a primary fuel source to power automobiles and homes, one of them being that unless it is in liquid form, which requires energy to maintain, hydrogen can take up a lot of volume and can be hard to store. Nevertheless, hydrogen is produced as a byproduct of nitrogen fixation in many bacteria. Nitrogen-fixing bacteria use nitrogenases to convert nitrogen gas into ammonia, a usable form of nitrogen to plants. Nitrogenases also reduce protons to hydrogen gas. R. palustris is able to produce nearly three times as much hydrogen as most other nitrogen-fixing bacteria because it codes for an enzyme called vanadium nitrogenase (Figure 5). Vanadium nitrogenase is found in members of the Azotobacter but is also found in the species R. palustris and Anabaena variabilis. This differs from the more common nitrogen-fixing enzyme, molybdenum nitrogenase, in its ability to reduce more protons and thus generate more hydrogen gas.
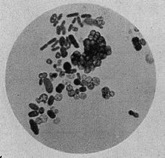
Electrical conductivity of biofilms
The formation of biofilms can play an important role in extracellular electron transfer. Previously, it was known that biofilms could only withhold insulation. But a recent study done by Malvankar et al. (2012) on Geobacter sulfurreducens shows shows that both mixed-species and pure culture biofilms have the potential for electrical conductivity. This is an important finding because it revealed that upon electron uptake, bacterium in a biofilm that were not in direct contact with an electrode contributed to current production equally as much as bacterium in direct contact. Thus, all bacterium in a biofilm have the potential to employ extracellular electron transfer.
Conclusion
An advance in our understanding of R. palustris and how it undergoes extracellular electron transfer has profound implications. Microbes that can perform electron extracellular transfer confers an ecological advantage – although soluble electron donors and acceptors are ubiquitous, the ability to acquire electrons from solid-phase materials can be beneficial in environments that are nutrient poor and uninhabitable to more basic microbes. Girguis’s study gave light to the possibility of using R. palustris in batteries or other practical appliances, although they were unsure that the bacterium would be a very efficient fuel source. If genes responsible for extracellular electron transfer were spliced into animal genomes or human genomes, could we acquire energy from our environment? This is a profound question that researchers are beginning to grapple with and explore.
References
1) Bose, A., Gardel, E.J., Girguis, P.R., Parra, E.A., Vidoudez, C., Electron uptake by iron-oxidizing phototrophic bacteria, Nature Communications, 2014, DOI: 10.1038/ncomms4391
2) Franks, A., Lau, J., Lovley, D., Malvankar, N., Nevin, K., Tuominen, M., Electrical Conductivity in a Mixed-Species Biofilm, Applied and Envrionmental Microbiology, 2012, Vol. 78, no.16 5967-5971.
3) http://en.wikipedia.org/wiki/Rhodopseudomonas_palustris
4) http://microbewiki.kenyon.edu/index.php/Rhodopseudomonas_palustris
5) http://en.wikipedia.org/wiki/Vanadium_nitrogenase