The Role of Antivirals in Influenza Treatment and Prevention
A Viral Biorealm page on the family The Role of Antivirals in Influenza Treatment and Prevention

Image source: http://www.nature.com/nsmb/journal/v17/n5/full/nsmb.1779.html [1]
Influenza virus A is the causative agent of seasonal influenza epidemics, and is responsible for thousands of deaths annually in the United States alone. New pandemic strains of influenza can arise via reassortment between human and animal-infecting influenza strains, and these pose an even greater risk to human health. For instance, the 1918 avian flu pandemic killed more than 40 million people worldwide [1]. The recent emergence of several zoonotic influenza strains, including the 2005 H5N1 (“bird flu”) and 2009 H1N1 (“swine flu”), demonstrates the constant threat of a deadly new pandemic [2]. Given the dangers posed by both seasonal and pandemic influenza outbreaks, it is important to continue developing prevention and treatment strategies.
Although annual vaccination programs successfully reduce the impact of seasonal influenza epidemics, these programs have significant limitations. The most obvious drawback is that they are preventative only, and offer no therapeutic use [4]. Furthermore, because it takes time to develop a vaccine to combat a newly emerging strain, vaccination programs are of limited use in preventing the initial spread of a new pandemic [1]. Antiviral drugs are an important complement to vaccination programs, because of both their use in influenza treatment, and their ability to inhibit the spread of new influenza strains. Although several antivirals are available clinically, the emergence of resistance in circulating viral strains highlights the need for the rapid identification of new drug targets and the development of new antiviral agents [4].
Overview of Influenza Virus
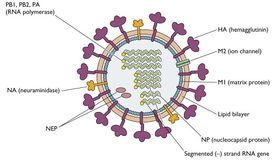
Image source: http://www.virology.ws/2009/04/30/structure-of-influenza-virus/
In order to understand how antiviral agents inhibit influenza, it is necessary to have a basic understanding of the virus’s characteristics and replication cycle (summarized in Figure 1).
Influenza virus is a member of the Orthomyxoviridae family of type V (-) RNA viruses. There are three genera of influenza viruses, including influenza A, B, and C, although B and C typically cause only local epidemics [3]. Because of its wide host range, influenza A poses a much greater risk to human health. The virus is capable of infecting humans, pigs, birds, and horses [15].
Virion Structure
The influenza virus has a segmented genome comprised of 8 distinct (-) RNA strands which synthesize 12 known polypeptides. Each strand is wrapped by a nucleoprotein (NP) trimer, and packaged with an RNA-dependent RNA polymerase [2]. The genome is contained along with other viral proteins by an enclosed layer of matrix proteins, which in turn is encapsulated by the viral envelope. The viral envelope is a cell-derived lipid bilayer, dotted with numerous viral surface proteins including neuraminidase, hemagglutinin, and M2 ion channel (M2) [1].
Viral Entry and Replication
The influenza surface protein HA mediates viral entry into the host cell by binding with a sialic acid-containing host glycoprotein, causing the virion to be taken up by endocytosis. The acidification of the endosome then triggers a conformational change in the sialic acid-bound HA, exposing the N-terminal fusion peptide. The viral envelope fuses with the endosome membrane, and the viral contents are released into the cell [9]. The (-) RNA genome enters the host cell nucleus, at which point RNA-dependent RNA polymerase begins synthesis of (+) mRNA, capping the transcripts by “snatching” caps from primed host mRNA. From this point forward, the virus subverts the host machinery for use in viral protein synthesis and genome replication [1].
Viral envelope proteins are synthesized at the endoplasmic reticulum, and then moved to the cell membrane via the golgi. Viral (-) RNA segments and proteins are packaged into capsids at the cell membrane. The loaded capsids then proceed to bud out of the cell, acquiring a viral-protein loaded envelope as they exit [1].
Antivirals Currently Used Against Influenza
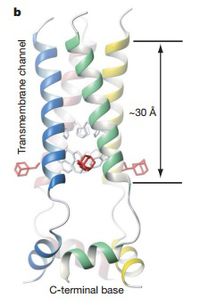
Image source: http://www.nature.com/nature/journal/v451/n7178/pdf/nature06531.pdf [5]
At the present, there are only two classes of antiviral drugs available for influenza treatment in the United States. These two types of drugs include M2 ion channel inhibitors (adamantane derivatives) and NA inhibitors [4].
M2 Ion Channel Inhibitors
The M2 ion channel is a viral transmembrane protein that spans the viral envelope and capsid. As shown in figure 3, M2 is a homotetramer with four transmembrane helices that form a pore in the influenza virion, allowing for the exchange of ions between the interior and exterior of the virus particle. The most important M2-mediated process occurs after the virus particle has been taken up by endocytosis, while the virion is still contained in an endosome (figure 1 part b). As the endosome is being acidified, M2 responds to the drop in pH by switching from a closed formation to an open formation. This allows for the free movement of protons between the virus particle and the endosome, thereby acidifying the virion interior. This internal pH change causes the matrix proteins to dissociate from the virus’s genetic material as the endosome membrane fuses with the envelope [6].
This dissociation of the matrix proteins is an essential step in the unpacking of the viral genome, and inhibiting this process prevents the infection from progressing. Two antiviral drugs, rimantadine and amantadine, function by this mechanism. These adamantane derivatives prevent the acidification of the viral interior by binding to M2 and stabilizing the protein’s closed conformation, thereby preventing it from switching to the open conformation. Rimantadine does this allosterically (figure 3), whereas the mechanism of amantadine is unknown. As a consequence of drug binding, the matrix proteins do not successfully dissociate, and viral unpacking does not proceed normally [5].
M2-inhibiting drugs used to be commonly prescribed for influenza treatment. However, in the last ten years circulating strains of influenza have evolved adamantane resistance. This resistance is now so commonplace in wild strains that neither amantadine nor rimantadine can effectively be used for influenza treatment. This rapid emergence of resistance both highlights the imminent need for new antiviral drugs, but should also serve as an example of the consequences of antiviral misuse [4].
Neuraminidase Inhibitors
NA is an influenza virus protein found on the envelope of the virion particle. The most important function of NA is its ability to cleave sialic acid- the same compound that HA binds in order to initiate virion entry. As the virus particles bud out of the host cell, HA interacts with sialic acid on the cell’s exterior, thereby preventing the virion from dispersing to infect a new cell. NA’s function is to cleave this sialic acid, preventing the aggregation of virions at the host cell membrane (figure 1 part g) [8].
The NA inhibitors available for clinical use are Oseltamivir (Tamiflu) and Zanamivir. Each of these compounds is structurally similar to sialic acid, and functions by competitively binding the active site of NA. Thus, by inhibiting the distribution of influenza virions these drugs successfully inhibit production of the virus [7].
Antiviral Developments: New Drug Targets and Compounds
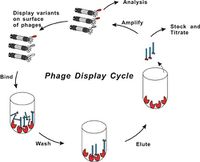
Image source: http://www.creative-biolabs.com/phagedisplay1.htm
Because of the constant threat of the emergence of antiviral-resistant influenza strains, it is important to continue the search for drugs that could be used for influenza prophylaxis and treatment. To this end, research continues to be done in order to identify new influenza antivirals that exploit a wider range of drug targets.
Hemagglutinin
The trimeric surface protein hemagglutinin presents an excellent drug target because it is critical for two steps of viral replication: it binds to sialic acid on the cell’s exterior membrane in order to induce endocytosis (figure 1 part a), and it also undergoes a conformational change when exposed to a lower pH, inducing membrane fusion after the virion-containing endosome is acidified (figure 1 part b). Because there are as many as 16 distinct recognized subtypes of HA, there is a challenge to identifying antivirals that would work against multiple subtypes. Nevertheless, a drug that competes with sialic acid for the HA binding site could theoretically work by preventing the virus from ever entering a host cell. In fact, a recent screen revealed two compounds that demonstrate potential for inhibiting HA subtype five [3].
However, most of the recent HA drug development research has focused on the protein’s membrane fusion function. For instance, the compound tert-butylhydroquinone (TBHQ) has been shown to bind three identical sites on the pre-fusion HA trimer, stabilizing the protein and preventing it from undergoing its conformational change when the pH decreases. Thus, TBHQ inhibits fusion of the viral and endosome membranes, thereby preventing viral replication. This compound shows promising potential: recent structural studies suggest that it might be possible to engineer a more effective TBHQ derivative to act as an influenza antiviral [9].
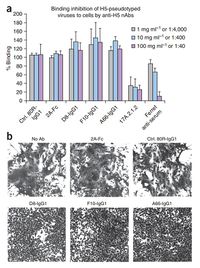
a) The presence of human antibodies D8, F10, or A66 does not inhibit binding of an H5-psuedotyped influenza virion to the sialic acid on the host cell membrane. 80R (an anti-SARS antibody) and 2A (an anti-HA1 monomer antibody) are known to not inhibit HA-5, and acted as negative controls. 17A.2.1.2 (an anti-H5 mouse antibody) is known to inhibit HA-5 binding, and acted as a positive control, along with ferret anti-serum.
b) A syncytia formation assay (see main text) demonstrates that 0.13 micromolar concentrations of human antibodies D8, F10, and A66 inhibit HA-5 facilitated membrane fusion. Treatments of no added antibody or equal concentrations of 2A or 80R act as controls.
Image source: http://www.nature.com/nsmb/journal/v16/n3/abs/nsmb.1566.html
Using Antibodies to Target Hemagglutinin
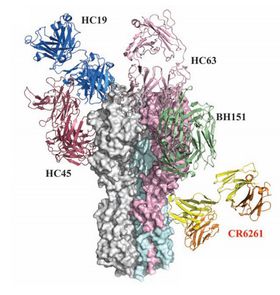
Image source: http://www.sciencemag.org/content/324/5924/246.full.pdf [10]
Hemagglutinin’s position on the surface of the influenza virion also makes it susceptible to recognition and binding by antibodies. Some studies have considered targeting HA by using neutralizing human antibodies (nABs), which work by binding some epitope of HA to prevent it from functioning correctly. Ordinarily this approach has limited success, because the protein's variable region is the most accessible part of the structure, and antibodies that target that region are of no use against other HA subtypes, and how to get around this natural variance is one of the biggest questions of this approach. Nevertheless, the research group of Sui et al. pursued this idea, and conducted a study to identify and characterize some of these potentially useful proteins.
At the start of their study, Sui et al. used a phage display technique to isolate nABs that bind to HA. This technique uses a library of mutant phages, each of which has a chimeric coat protein that presents a segment of a human antibody. These antibody-expressing phages are then run over a bound layer of cells engineered to express trimeric HA subtype 5 on their cell membranes. The phages expressing antibodies that successfully bind to the HA “stick” to the layer of cells via antibody/HA interactions, while the phages with “incorrect” antibodies are washed away. The phages expressing the desirable antibodies are then isolated and amplified, and their DNA is sequenced to identify the antibody of interest. Through this technique, Sui et al identified ten different anti-HA antibodies. The researchers proceeded to confirm that the antibodies could neutralize H5N1, and then went on to test three of their antibodies in a mouse model. They demonstrated that 80% to 100% of mice treated with one of the antibodies between 1 hour before and 72 hours after H5N1 infection survived, compared to control rates of 0% to 20% survival.
Sui et al. also investigated the mechanism of action of the antibodies: they found that none of their isolated antibodies inhibited binding of influenza virus particles to sialic acid on cell surfaces. They followed up on this result by investigating whether or not the antibodies were capable of inhibiting fusion, by transfecting HeLa cells with an HA subtype 5-containing plasmid, and then exposing the cells to a pH 5.0 buffer in the presence or absence of the antibody. In the absence of the nABs, the low pH conditions induced the HA on the surface of the transfected cells to undergo its conformational change, revealing the fusion peptide and inducing fusion of different cells’ membranes. This resulted in the visible formation of masses of multinucleate cells. However, these multinucleate cells did not form in the presence of the nABs, implying that their antibodies were capable of inhibiting HA-5’s fusion functionality (Figure XXX).
Thus, whereas the vast majority of anti-HA human antibodies work by targeting the variable “head” region of the protein that binds sialic acid, the antibodies isolated in this study work by inhibiting HA’s role in fusion. Structural analyses revealed that they do so by binding the fusion peptide, which is concealed in the conserved “stem” region at the base of the protein. Binding this region prevents the protein from undergoing the pH-induced conformational change, thereby inhibiting viral entry and replication. This unusual characteristic of their nABs is likely a result of an abnormal presentation of HA during their phage display protocol, which might have revealed the usually hidden fusion peptide. Because this region is conserved across a wide variety of HA subtypes, these antibodies are capable of inhibiting a wide variety of HA subtypes, thereby overcoming the greatest barrier to using HA as a drug target. Sui et al. confirmed this by demonstrating that one of their antibodies, F10, could also neutralize viruses with HA subtypes 1, 2, 6, 8, 9, and 11.
Ekiert et al. isolated a different antibody, CR6261, that acts against in the same way. Because of their ability to target so many HA subtypes, these human antibodies appear to be promising new candidates for antiviral drugs. And even in the case of complications with immunotherapy, the structures of F10 and CR6261 can be used as tools for rational drug design to produce compounds that will work.
Nucleoprotein
The function of nucleoprotein (NP) is to form a trimer to coat viral mRNA and bind with viral RNA polymerase in order to facilitate the replication of viral RNA [14]. The protein also has an important role in the transcription of viral RNA, and the loading of genetic material into the virions [3]. Because NP is involved in so many integral processes of viral replication, it has recently been considered as a anti-influenza drug target [13].
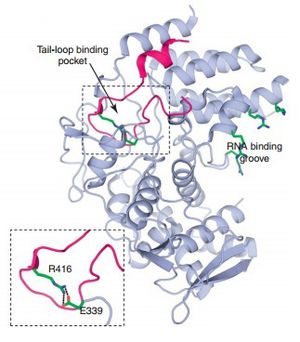
Image source: http://journals.ohiolink.edu/ejc/pdf.cgi/Du_J.pdf?issn=13596446&issue=v17i19-20&article=1111_rpisadd [3]
NP contains three distinct binding regions which allow it to form the trimer that coats RNA. The first is the RNA-binding groove, the surface of the protein that interacts with viral RNA. The second is the tail-loop, a peptide strand that sticks out from the main body of the protein and interacts with a neighboring NP. The third region is the tail-loop pocket, a cavity in the protein that accepts the tail-loop of another NP (figure 5). The tail-loop and the pocket have both been considered as potential drug targets, because blocking their interaction would prevent the NP trimer from forming, and therefore would inhibit the core processes of viral replication [3].
Kao et al. recently identified a compound called nucleozin via random screening, which was found to inhibit influenza by interacting with influenza NP. Nucleozin causes the NPs to aggregate abnormally, and consequently inhibits normal viral transcription, crippling the replication cycle by extension [12]. Examination of a nucleozin analogue revealed that the compound functions by binding to two copies of NP and forming abnormal dimers, causing the proteins to aggregate and preventing them from functioning normally [13]. Nucleozin was also shown to inhibit influenza virus in vitro and in a mouse model, making it a promising candidate for a new antiviral drug [12].
Rational Drug Design: The Tail-Loop Binding Pocket
The identification of nucleozin and subsequent nucleozin-like compounds represents significant progress in the search for anti-influenza drugs. However, mutations that confer resistance to nucleozin have already been identified. This find demonstrates how it is always possible for resistance to emerge, emphasizing the need to continue seeking out compounds that work against NP in different ways. Shen et al. have approached this problem from a rational drug design perspective, taking advantage of the idea that they might be able to use NP structural information to identify (or design) a compound that could bind an important site on the protein, and therefore sterically inhibit some important function.
Prior to identifying compounds, Shen et al. demonstrated that replacing one of two important NP residues, glutamic acid 399 or arginine 416, with alanine both prevents NP subunits from binding with each other and inhibits viral replication. These residues are sensitive to mutations because they combine in the tail-loop binding pocket to form a critical salt bridge, which is essential for forming a sturdy connection between NP monomers. Because this salt bridge is so important, they hypothesized that a compound with the ability to disrupt this connection might be an effective antiviral. To seek proof of concept for their idea, they investigated whether a tail-loop peptide (without the rest of the NP) would bind in the pocket and prevent the NP subunits from binding with each other by competitively occupying the space. In order to answer this question, they transfected human embryonic kidney cells with either a simple green fluorescent protein (GFP) plasmid, or a GFP-Tail Loop plasmid, and then infected the cells with an H1N1 strain. They found that the presence of the tail-loop peptide reduced synthesis of viral proteins and decreased virion production (figure XXX), which suggested that it might be possible to do the same with a different compound.
Shen et al. then proceeded to use a virtual screening technique to identify known compounds from a library that would fit the NP tail-loop binding pocket both structurally and electrostatically. Their screening method revealed 24 hits, from which they identified four effective compounds. All four of these compounds were shown to be able to provide MDCK cells with some degree of protection from H1N1, and were able to reduce viral transcription activity in vitro. The authors used immunofluorescence to visualize NP distribution throughout compound-exposed infected cells. A comparison of “compound 3”-exposed infected cells with nucleozin-exposed infected cells demonstrated that this new compounds do not cause NP to aggregate like nucleozin and its analogous do, providing evidence that these new compounds function via a different mechanism. Thus, Shen et all successfully identified several new anti-influenza compounds, all of which could go on to be optimized during drug development. This study illustrates the utility of rational drug design, and how the continued study of influenza proteins can lead directly to developments in the search for antivirals.
Potential Future Drug Targets
Structural information is now available for many influenza proteins, and the continued analysis of their different binding domains could potentially lead to their use as novel drug targets. Although many viral proteins fit into this category, including matrix protein 1, nuclear export protein, and non-structural protein 1, one of the more promising potential targets is RNA polymerase.
RNA-Dependent RNA Polymerase
Influenza’s viral RNA polymerase is a trimer, made up of viral proteins PB1, PB2, and PA. Its primary function is to use its RNA-dependent RNA synthesis function to create (+) sense viral mRNAs, and also to replicate the (-) sense viral genome. The viral polymerase is also responsible for capping the mRNAs that it synthesizes with a 5’ methyl guanosine cap, which it does by “cap snatching”, the cleaving and stealing of 5’ caps from cellular mRNAs for use in capping viral mRNAs.
Viral RNA polymerase has several different domains which it needs to carry out all of its functions, including a polymerase domain, an RNA binding domain, a cap-binding domain, an endonuclease domain, and more. This wide array of potential drug-binding sites, coupled with the importance of the protein, make this very appealing for drug target research. There are signs that it could be targeted in this way in the near future: the research group of Iwai et al. recently identified the compound “marchantin E” by random screening. They demonstrated that this compound is capable of inhibiting viral RNA polymerase endonuclease activity in vitro, which would prevent the protein from cap snatching, and therefore prevent viral RNAs from being translated. As further confirmation, the authors found that marchantin E is capable of inhibiting growth of H3N2 and H1N1 viruses. This recent discovery might yield new avenues of research into viral polymerase as a drug target, and potentially lead to an all new type of influenza antiviral.
References
[1] Das K, Aramini JM, Ma L, Krug RM, Arnold E. 2010. Structures of influenza A proteins and insights into antiviral drug targets. Nat Struct Mol Biol 17:530-8.
[2] Das K. 2012. Antivirals Targeting Influenza A Virus. J Med Chem 55(14):6263-77.
[3] Du J, Cross TA, Zhou H. 2012. Recent progress in structure-based anti-influenza drug design. Drug Discov Today 17(19-20):1111-20.
[4] Poland GA, Jacobson RM, Ovsyannikova IG. 2009. Influenza Virus Resistance to Antiviral Agents: A Plea for Rational Use. Clin Infect Dis 48(9):1254-56.
[5] Schnell JR, Chou JJ. 2008. Structure and mechanism of the M2 proton channel of influenza A virus. Nature 451:591-6.
[6] Cady SD, Luo W, Hu F, Hong M. 2009. Structure and Function of the Influenza A M2 Proton Channel. Biochem 48(31):7356-64.
[7] Collins PJ, Haire LF, Lin YP, Liu J, Russell RJ, Walker PA, Skehel JJ, Martin SR, Hay AJ, Gamblin SJ. 2008. Crystal structures of oseltamivir-resistant influenza virus neuraminidase mutants. Nature 453:1258-62.
[8] Wagner R, Matrosovish M, Klenk H. 2002. Functional balance between haemagglutinin and neuraminidase in influenza virus infections. Rev Med Virol 12:159-66.
[9] Russell RJ, Kerry PS, Stevens DJ, Steinhauer DA, Martin SR, Gamblin SJ, Skehel JJ. 2008. Structure of influenza hemagglutinin in complex with an inhibitor of membrane fusion. Proc Natl Acad Sci 105(46):17736-41.
[10] Ekiert DC, Bhabha G, Elsliger M, Friesen RHE, Jongeneelen M, Throsby M, Goudsmit J, Wilson IA. 2009. Antibody Recognition of a Highly Conserved Influenza Virus Epitope. Science 324:246-51.
[11] Sui J, Hwang WC, Perez S et al. 2009. Structural and functional bases for broad-spectrum neutralization of avian and human influenza A viruses. Nat Struct Mol Biol 16(3):265-73.
[12] Kao RY, Yang D, Lau L et al. 2010 Identification of influenza A nucleoprotein as an antiviral target. Nat Biotech 28(6):600-7.
[13] Gerritz SW, Cianci C, Kim S et al. 2012. Inhibition of influenza virus replication via small molecules that induce formation of higher-order nucleoprotein oligomers. Proc Natl Acad Sci 109(46):15366-71.
[14] Shen Y, Chen Y, Chu S et al. 2011. E339...R416 salt bridge of nucleoprotein as a feasible target for influenza virus inhibitors. Proc Natl Acad Sci 108(40):16515-16520.
[15] Suzuki Y, Ito T, Suzuki T, Holland RE, Chambers TM, Kiso M, Ishida H, Kawaoka Y. 2000. Sialic Acid Species as a Determinant of the Host Range of Influenza a Viruses. J Virol 74(24):11825-31.
Page authored for BIOL 375 Virology, September 2010