Coxsackievirus Type B

Classification
Higher Order Taxa and Species Viruses, ssRNA viruses, ssRNA positive-strand viruses, no DNA stage, Picornavirales, Picornaviridae, Enterovirus, Enterovirus B (1).
Subtype
There are six different serotypes of Coxsackievirus B (CVB) 1-6 (2).
Introduction
Coxsackievirus Type B (CVB) is a member of the Picornavirus family, Enteroviridae genus (1-6). The viral packaging and capsid structure is conserved across this class of viruses, diverging only at the protein sequence level (1-8). CVB causes a variety of diseases and symptoms in diverse tissues; from mild, cold-like symptoms, to severe, inflammatory myocarditis (9) and diabetes mellitus (10). Many studies investigate the signal transduction pathways stimulated upon viral docking, entry, and formation (11-50). This converges into a response that is detrimental to cell and tissue homeostasis. Sufficient understanding of the molecular mechanisms behind the CVB life cycle is critical to continual development of safe, effective, and specific antiviral therapies (51-57).
Virus Structure and Genome
CVB consists of two parts: a capsid covering and single-stranded RNA genome (1, 2). The genome is made of approximately 7400 nucleotides encoding a 250 kDa polyprotein. The genome can be separated into three regions: P1, P2, and P3. P1 encodes for structural proteins that form the capsid, and P2 and P3 encode nonstructural proteins for viral replication (2).
The capsid of CVB is made of protein subunits VP0 through VP4 (1). VP1, VP2, and VP3 are called the “B sandwich”. These subunits are β sheets that are antiparallel and layered on top of each other (5). The most prominent loop in between the β sheets consists of 12 amino acids that stabilizes the structure of CVB and allow it to survive in the acidic gut. To prevent recognition from the host, the CVB structure contains a BC loop, β strands in a helical pattern, in VP1 and EF Loop, and a helix-loop-helix structure, in VP2. EF loop functions for CVB recognition or binding region. VP3 subunit forms a B barrel, B sheets lined next to each other in a ring formation that is conserved across species in the enterovirus genus (5). Under VP3 subunit is a myristate fatty acid, attached to VP4 that stabilizes the structure during virus construction (6). On the exterior of the capsid is a fivefold channel that is involved with virus deconstruction.
Virus Evolution
CVB sequence is more similar to polioviruses than rhinoviruses (5). When comparing the sequences of the six most conserved proteins in the family Picornavirdae, the viruses are placed into groups at three hierarchical levels (7). Enteroviruses are closest to Sapeloviruses on the phylogenetic tree (7). CVB is monophyletic relative to other enterovirus species except in the 5’ untranslated region (8). The 5’ untranslated region that determines the viruses’ infection severity is conserved across CVB strains (8, 11). In this region, CVB is clustered with members of Coxsackievirus type A (CVA) and is least similar to Coxsackievirus type C (CVC) and D (CVD) (8). Therefore, CVB is more closely related to one another than they are to CVC or CVD. The most conserved protein sequence for CVB is the “β sandwich” and VP3 areas. Compared to other viruses, the sequence of the VP1 region and cleavage protein 2A differs the most (2, 5).
Virus Entry
CVB entry into cells is regulated at the viral receptor interface and is mediated by intracellular signal cascades (9, 12-14). Entry is dependent upon binding to Coxsackievirus Adenovirus Receptor (CAR), which assists in viral conformational change and endocytosis (15-18). Once in the host extracellular environment, virus entry mechanisms depend on cell polarity.
In polarized cells, such as epithelial cells that have two heterogeneous, dissimilar, opposing membranes, the CAR is on tight junctions (TJ) where cells contact each other, and far from the apical surface where CVB is present (9, 14, 17). CVB translocates to the TJ by the Decay Accelerating Factor (DAF) protein. At the apical surface, DAF binds to the CVB and triggers a signal cascade regulated by Abl kinase and Rac1, which modulate actin dynamics (15, 17). Actin facilitates DAF-CVB translocation to the polarized TJs, then complexes with CAR to form 135S A particles (15, 17, 18). DAF-CVB also activates Fyn kinase, causing Cav1 phosphorylation (15, 17, 19). Cav1 phosphorylation triggers internalization of CVB through caveosomes at the cell membrane and TJs (15, 17, 20).
In nonpolarized cells, such as HeLa cells with homogenous membranes, the same type of receptor-mediated signaling and entry occur but CAR is already present in the extracellular environment causing direct binding of the CVB with CAR (15, 20). DAF is still present for CVB binding and can enhance CAR-CVB complex formation (15, 20-22). Entry results in further uncoating processes by the viral capsid, until the viral genome is released into the cytoplasm for subsequent replication (15). During this process, many cell signaling axes activated through viral hijacking, to either enhance pathogenesis or diminish the spread of CVB infection.
The CVB-DAF-CAR interactions complex mechanisms that induce internalization, uncoating, and viral release, leading to eventual disruption to other host cell signaling pathways.
Virus and Intracellular Host Signaling

CVB infection hijacks many host signaling pathways to produce progeny, and lyse the cell. These effects point to a global picture of cellular dysfunction from CVB infection.
Caspase Activation and Apoptosis
Caspase cleavage and activation of apoptosis pathways is necessary for viral release, occurring late in the virus life cycle. Apoptosis is caspase-dependent (22). After CVB3 infection, caspases are cleaved in response to cytochrome c release from the mitochondria to begin programmed death (23). However, expression of anti-apoptotic factors alone does not fully rescue the cell death response and viral release (23). Glycogen Synthase Kinase 3β (GSK3β) can also regulate apoptotic, caspase-dependent responses through β-catenin phosphorylation and inhibition to encourage CVB progeny release (24). Together, combined signaling pathways lead to cell death for viral release.
Matrix Metalloproteases (MMPs)
Matrix Metalloproteases (MMPs) are enzymes that digest the extracellular matrix (ECM), a prerequisite for removal of dead or dying cells and activation of signaling cytokines. After CVB infection, MMPs are upregulated in tissues, such as the heart (25). MMPs controls the viral response post infection, both at the transcriptional and extracellular/posttranslational levels (25). Ultimately, it is important for balancing cell clearance and systemic antiviral responses during CVB infections (25).
Extracellular Regulated Kinases (ERKs)
CVB3 infection induces phosphorylation of Extracellular Regulated Kinases (ERKs) 1/2 early and late after infection (26). ERK1/2 controls viral progeny and caspase production. Receptor-mediated signaling regulates early phase ERK activity (26). Positive feedback mechanisms involving viral production and ERK phosphorylation are required for late phase activity (26, 27). CVB3 infection simultaneously increases p38 activation, which induces ERK5, and inhibits ERK1/2, thereby partially blocking apoptosis. ERK inhibition of apoptosis and p38 induction of necrosis facilitates maximal viral progeny release (28).
Rac-Alpha Serine/Threonine Protein Kinase (Akt)
The Phosphoinositide 3 Kinase-Rac-Alpha Serine/Threonine Protein Kinase (PI3K-Akt) signaling axis plays a role in propagating CVB. CVB3 infection induces PI3K dependent Akt phosphorylation (30). Akt activation subsequently controls GSK3β phosphorylation and inhibition, further regulating CVB mediated GSK3β activity (25). PI3K-Akt activation is dependent on post entry viral replication/production and propagates the formation of progeny (28). Akt activation is also dependent on Integrin-Linked Kinase (ILK). ILK activity is increased after CVB infection, mediating signals for viral production (31). The results point to a combined PI3K-ILK-Akt axis as an important component of viral production.
Stress-Activated Protein Kinases (SAPKs)
Stress-activated protein kinases (SAPKs) induce apoptotic pathways when cell damage is irreversible, and are necessary for attenuating viral pathogenicity. After CVB3 infection, c-Jun N-Terminal Kinases (JNKs) 1/2 are activated. JNK is upregulated during SAPKs responses and has minor roles in CVB production (32). Notably, JNK1/2 controls the expression of protein Cyr61, which promotes CVB production and cell death for viral release (33). An additional SAPK is the Janus Kinase (JAK), in combination with the effector Signal Transducer and Activator of Transcription (STAT). The JAK-STAT pathway is important for inducing the protective antiviral response. This pathway is inhibited by Suppressor of Cytokine Signaling (SOCS), increasing the amount of viral progeny released (34). Integrated SAPKs activity can be an inhibitor of viral propagation and protector of cell viability.
Global Overview
Overall, CVB can perturb these pathways simultaneously. One small network model implicates IκBα as the hub and connects Akt, p38, JNK, ERK, GSK3β, and ATF-2 (35). IκBα is regulated by pro-inflammatory cytokines, TNF and IL-1, which induce cell death and viral release, highlighting its importance in CVB infection (35). Direct modulation of the above-described pathways during the course of the viral life cycle enables viral toxicity and production, further exacerbating infection in surrounding tissues (36-38). The inevitable end point for this toxic response is usually organ failure and death if the viral pathogen is not quickly eliminated. Without the proper function of critical signaling pathways, such as ERK, MAPKs, PI3K-Akt, and SAPKs, the host cell is unable to prevent further viral pathogenesis.
Viral Replication
Although the complete mechanism of CVB replication is yet to be elucidated, CVB replication is known to proceed through the following steps: viral protein translation, synthesis of negative-strand RNA, and replication of positive strand RNA. Following viral entry into the host cell cytoplasm, viral RNA undergoes translation using the host cell translational machinery (36). The CVB RNA encodes for a 250 kDa polyprotein that is processed into mature viral proteins by its own endopeptidases, 2A and 2C. Host cell cap-dependent protein translation is inhibited by the viral 3C and 2A proteins (37). The 3A protein recruits a lipid modifying enzyme, phosphatidylinositol-4-kinase IIIβ (PI4KIIIβ), which allows the formation of a replication organelle (38-40). The negative strand synthesis involves the formation of circular ribonucleoprotein complex mediated by binding of viral 3CD protein, host cell poly (rC) binding protein (PCBP2), and poly (A) binding protein (PABP) on the RNA. The uridylylated VPg protein serves as a primer for 3D polymerase to synthesize the negative RNA strand from the 3’ end (41-43). The completion of the negative-strand synthesis and the denaturation of duplex RNA initiates positive-strand synthesis which involves the uridylylated VPg, 3D Pol, 2C, and 3CD viral proteins, as well as the host cell heterogeneous ribonuclear protein C (38, 44, 45). CVB is known to be highly lytic, however, there are emerging evidences that support the existence of non-lytic dissemination mechanisms (46). After the newly synthesized positive-strand RNA are packaged into virions, they are sequestered in autophagosome-like organelles, budding from the plasma membrane as microvesicles (47-50). CVB continues to infect cells until the virus reaches a latent stage, when the virus is not actively replicated, or gets eliminated by the immune system (46, 58).
Immune System Response and Autoimmunity
The body’s innate and adaptive immune systems react to CVB infection. In the innate immune system, CVB interacts with Toll-like receptors (TLR), which are transmembrane glycoproteins (58). The extracellular TLR4 interacts with the CVB and the intracellular TLR3 recognizes viral replication (58). However, the viral genome-linked protein (VPg protein) which is bound to the 5’ viral RNA, prevents detection by the RIG-I-like receptor (58). This causes a cascade response by the innate immune system (58). Mitochondrial antiviral signaling also helps to fight off the CVB infections by killing host cells (58). Natural Killer (NK) cells help prevent pancreatitis and myocarditis by limiting CVB viral replication (58). When the innate immune response is unable to clear the infection, the adaptive immune response is activated. B cells, which are not processed by the thymus gland, rapidly respond to CBV infection by first producing Immunoglobulin M (IgM) and Immunoglobulin G (IgG) antibodies. These induce response from CD4 T cells, which are processed by the thymus gland. IgM and IgG enlist B Cells and CD8 T Cells to kill infected cells (58). CVB inhibits the major histocompatibility complex (MHC) class I receptors on the cells, which present antigens to T and B cells, preventing recognition of infection (58). In turn, low antigen expression prevents the activation of naïve T cells, which can be offset by the presence of memory T Cells for CVB (58). CVB infection may result in autoimmunity, particularly in children, and increases the risk of developing Type I Diabetes (10).
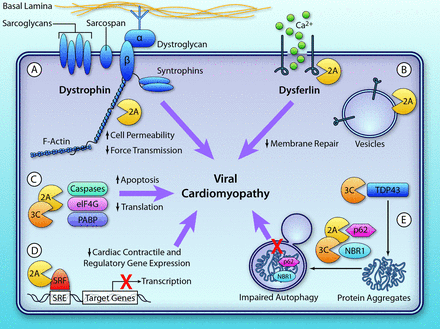
Viral Induced Diseases
The damage caused by CVB is extensive. The basis of CVB induced disease is due to both extreme damage in a singular tissue, as well as chronic persistence of the virus. Some of these include Myocarditis and Diabetes.
Myocarditis Implications
Myocarditis is the inflammation of myocardium tissue, with simultaneous infiltration by mononuclear immune cells (9). Acute or chronic infections exist, and the area can be diffuse or focal depending on the extent of immune cell infiltration (59). CVB infection and subsequent hijacking of the aforementioned signaling pathways induce cell toxicity (9, 12-14, 59). Furthermore, the viral proteases such as VP2A, directly target and cleave cell substrates important for host transcription, translation, and structure, further exacerbating cell homeostasis (13-14, 59, 61). In cardiomyocytes, this includes cleavage of dystrophin, which directly disrupts the dystrophin-glycoprotein complex (DGC), and leads to weakening of the sarcolemma membrane (12, 59, 62). This direct damage facilitates targeted viral production and cellular apoptosis/necrosis for efficient viral release. The balance of immune cells initiating a robust antiviral response without further tissue destruction is imperative to adaptive immunity and preserved tissue function (9, 12, 14, 59). Continual cellular dysfunction and activation of MMPs destroys the surrounding heart tissue, leading to compensation with possible fibrosis and dilated cardiomyopathy (DCM) (13, 60). DCM can occur due to persistent viral genomes and VP2A expression, implicating chronic infection as an underlying cause of DCM (61-63). Thus robust therapies that preserve heart tissue are important to counteract CVB myocardial infections.
Diabetes Implications
Some CVB serotypes correlate with the formation of Type 1 diabetes (10). Type 1 diabetes is the pancreas’ inability or limited ability to form insulin, due to whole or partial loss of function. The entry of CVB into the bloodstream can lead to selective infection in the pancreas and thymus (64). The thymus infection prevents the cell from distinguishing self from nonself cells, resulting in autoimmunity against organs, such as the pancreas (64). Infection in the pancreas causes bursts in pancreatic cells. Consequence of both infections is type 1 diabetes, mostly due to thymus infections (64).
Antiviral Therapy
There are currently no effective, approved drugs against CVB. Pleconaril, a viral capsid inhibitor, has been rejected by the US Food and Drug Administration due to the lack of drug safety information (51). This inhibitor is known to cause adverse drug interactions by increasing cytochrome P450 activity (52) and give rise to viral drug resistance (53). Much of the current research focuses on identifying other therapies. For example, pyrazolopyrimidines, a novel capsid inhibitor, currently has no adverse effects and is effective in treating myocarditis in mice (54). Other possible therapeutic methods include the use of soluble Coxsackievirus and Adenovirus Receptor (CAR) as decoys to competitively inhibit the host cell surface receptors (55). Efforts towards producing a RNA vaccine against CVB is also in progress (56), and work is required to prove their therapeutic potential (57). General research is ongoing (65).
References
1. Martino T., Petric, M., Weingartl, H., Bergelson, J.M., Opavsky, M.A., Richardson, C.D., Modlin, J.F., Finberg, R.W., Kain, K.C., Willis, N. and Gauntt, C.J. 2000. The Coxsackie-Adenovirus Receptor (CAR) Is Used by Reference Strains and Clinical Isolates Representing All Six Serotypes of Coxsackievirus Group B and by Swine Vesicular Disease Virus. Virology, 271: 99–108.
2. Hughes, M., Hoey E., and Coyle P. 1993. A nucleotide sequence comparison of coxsackievirus B4 isolates from aquatic samples and clinical specimens. Epidemiol Infect., 110(2): 389–398.
3. Melnick, J. 1990. Enteroviruses, polioviruses, coxsackie viruses, echo viruses, and newer enteroviruses. Virology 2: 549-605. Raven Press Ltd. New York.
4. He, Y., Chipman, P.R., Howitt, J., Bator, C.M., Whitt, M.A., Baker, T.S., Kuhn, R.J., Anderson, C.W., Freimuth, P. and Rossmann, M.G. 2001. Interaction of coxsackievirus B3 with the full length coxsackievirus-adenovirus receptor. Nature structural biology, 8(10): 874.
5. Muckelbauer, J.K., Kremer, M., Minor, I., Diana, G., Dutko, F.J., Groarke, J., Pevear, D.C. and Rossmann, M.G. 1995. The structure of coxsackievirus B3 at 3.5 Å resolution. Structure, 3(7): 653-667.
6. Ansardi, D., Porter, D. and Morrow, C. (1992). Myristylation of poliovirus capsid precursor P 1 is required for assembly of subviral particles. J. Virol., 66: 4556-4563.
7. Lauber, C. and Gorbalenya, A. Toward Genetics-Based Virus Taxonomy: Comparative Analysis of a Genetics-Based Classification and the Taxonomy of Picornaviruses. 2012. J. Virol, 86(7): 3905-3915.
8. Oberste, M., Maher, K., and Pallansch, M. 2004. Evidence for frequent recombination within species human enterovirus B based on complete genomic sequences of all thirty-seven serotypes. J. Virol, 78(2): 855-86.
9. Yajima, T. and Knowlton, K., 2009. Viral myocarditis. Circulation, 119(19): 2615-2624.
10. Laitinen, O.H., Honkanen, H., Pakkanen, O., Oikarinen, S., Hankaniemi, M.M., Huhtala, H., Ruokoranta, T., Lecouturier, V., André, P., Harju, R. and Virtanen, S.M. 2013. Coxsackievirus B1 is associated with induction of β-cell autoimmunity that portends type 1 diabetes. Diabetes, 63(2): 446-55.
11. Liu, B., Li, F., Xiang, F., Wang, G., Zheng, Y. and Li, Z. 2014. The Whole Genome Sequence of Coxsackievirus B3 MKP Strain Leading to Myocarditis and Its Molecular Phylogenetic Analysis. Virology Journal, 11: 33.
12. Knowlton, K. 2008. CVB infection and mechanisms of viral cardiomyopathy. Current topics in microbiology and immunology, 323: 315-336.
13. Marchant, D., Si, X., Luo, H., McManus, B. and Yang, D. 2008. The impact of CVB3 infection on host cell biology. Group B Coxsackieviruses: 177-198.
14. Esfandiarei, M. and McManus, B. 2008. Molecular biology and pathogenesis of viral myocarditis. Annu. Rev. pathmechdis. Mech. Dis., 3:127-155.
15. Marjomäki, V., Turkki, P., and Huttunen, M. 2015. Infectious entry pathway of enterovirus B species. Viruses, 7(12): 6387-6399.
16. Shi, Y., Chen, C., Lisewski, U., Wrackmeyer, U., Radke, M., Westermann, D., Sauter, M., Tschöpe, C., Poller, W., Klingel, K. and Gotthardt, M. 2009. Cardiac deletion of the Coxsackievirus-adenovirus receptor abolishes Coxsackievirus B3 infection and prevents myocarditis in vivo. Journal of the American College of Cardiology, 53(14): 1219-1226.
17. Coyne, C and Bergelson, J. 2006. Virus-induced Abl and Fyn kinase signals permit Coxsackievirus entry through epithelial tight junctions. Cell, 124(1): 119-131.
18. Milstone, A., Petrella, J., Sanchez, M.D., Mahmud, M., Whitbeck, J.C. and Bergelson, J.M. 2005. Interaction with coxsackievirus and adenovirus receptor, but not with decay-accelerating factor (DAF), induces A-particle formation in a DAF-binding coxsackievirus B3 isolate. J. Virol, 79(1): 655-660.
19. Coyne, C., Shen, L., Turner, J., and Bergelson, J., 2007. Coxsackievirus entry across epithelial tight junctions requires occludin and the small GTPases Rab34 and Rab5. Cell host & microbe, 2(3): 181-192.
20. Patel, K., Coyne, C. and Bergelson, J., 2009. Dynamin-and lipid raft-dependent entry of decay-accelerating factor (DAF)-binding and non-DAF-binding coxsackieviruses into nonpolarized cells. J. Virol, 83(21): 11064-11077.
21. Nicholson-Weller, A. and Wang, C., 1994. Structure and function of decay accelerating factor CD55. The Journal of laboratory and clinical medicine, 123(4): 485-491.
22. Lublin, D. and Atkinson, J., 1989. Decay-accelerating factor: biochemistry, molecular biology, and function. Annual review of immunology, 7(1): 35-58.
23. Yuan, J.P., Wei, Z.H.A.O., Wang, H.T., Wu, K.Y., Tao, L.I., Guo, X.K. and Tong, S.Q. 2003. Coxsackievirus B3-induced apoptosis and caspase-3. Cell research, 13(3): 203-209.
24. Carthy, C.M., Yanagawa, B., Luo, H., Granville, D.J., Yang, D., Cheung, P., Cheung, C., Esfandiarei, M., Rudin, C.M., Thompson, C.B. and Hunt, D.W. 2003. Bcl-2 and Bcl-xL overexpression inhibits cytochrome c release, activation of multiple caspases, and virus release following coxsackievirus B3 infection. Virology, 313(1): 147-157.
25. Yuan, J., Zhang, J., Wong, B.W., Si, X., Wong, J., Yang, D. and Luo, H. 2005. Inhibition of glycogen synthase kinase 3β suppresses coxsackievirus-induced cytopathic effect and apoptosis via stabilization of β-catenin. Cell Death & Differentiation, 12(8).
26. Marchant, D.J., Bellac, C.L., Moraes, T.J., Wadsworth, S.J., Dufour, A., Butler, G.S., Bilawchuk, L.M., Hendry, R.G., Robertson, A.G., Cheung, C.T. and Ng, J. 2014. A new transcriptional role for matrix metalloproteinase-12 in antiviral immunity. Nature medicine, 20(5): 493-502.
27. Luo, H., Yanagawa, B., Zhang, J., Luo, Z., Zhang, M., Esfandiarei, M., Carthy, C., Wilson, J.E., Yang, D. and McManus, B.M. 2002. Coxsackievirus B3 replication is reduced by inhibition of the extracellular signal-regulated kinase (ERK) signaling pathway. J. Virol, 76(7): 3365-3373.
28. Lim, B.K., Nam, J.H., Gil, C.O., Yun, S.H., Choi, J.H., Kim, D.K. and Jeon, E.S. 2005. Coxsackievirus B3 replication is related to activation of the late extracellular signal-regulated kinase (ERK) signal. Virus research, 113(2): 153-157.
29. Jensen, K.J., Garmaroudi, F.S., Zhang, J., Lin, J., Boroomand, S., Zhang, M., Luo, Z., Yang, D., Luo, H., McManus, B.M. and Janes, K.A. 2013. An ERK-p38 subnetwork coordinates host cell apoptosis and necrosis during coxsackievirus B3 infection. Cell host & microbe, 13(1): 67-76.
30. Esfandiarei, M., Luo, H., Yanagawa, B., Suarez, A., Dabiri, D., Zhang, J. and McManus, B.M. 2004. Protein kinase B/Akt regulates coxsackievirus B3 replication through a mechanism which is not caspase dependent. J. Virol, 78(8): 4289-4298.
31. Esfandiarei, M., Suarez, A., Amaral, A., Si, X., Rahmani, M., Dedhar, S. and McManus, B.M. 2006. Novel role for integrin-linked kinase in modulation of coxsackievirus B3 replication and virus-induced cardiomyocyte injury. Circulation research, 99(4): 354-361.
32. Si, X., Luo, H., Morgan, A., Zhang, J., Wong, J., Yuan, J., Esfandiarei, M., Gao, G., Cheung, C. and McManus, B.M. 2005. Stress-activated protein kinases are involved in coxsackievirus B3 viral progeny release. Journal of virology, 79(22): 13875-13881.
33. Kim, S.M., Park, J.H., Chung, S.K., Kim, J.Y., Hwang, H.Y., Chung, K.C., Jo, I., Park, S.I. and Nam, J.H. 2004. Coxsackievirus B3 infection induces cyr61 activation via JNK to mediate cell death. Journal of virology, 78(24): 13479-13488.
34. Yasukawa, H., Yajima, T., Duplain, H., Iwatate, M., Kido, M., Hoshijima, M., Weitzman, M.D., Nakamura, T., Woodard, S., Xiong, D. and Yoshimura, A. 2003. The suppressor of cytokine signaling–1 (SOCS1) is a novel therapeutic target for enterovirus-induced cardiac injury. Journal of Clinical Investigation, 111(4): 469.
35. Garmaroudi, F.S., Marchant, D., Si, X., Khalili, A., Bashashati, A., Wong, B.W., Tabet, A., Ng, R.T., Murphy, K., Luo, H. and Janes, K.A. 2010. Pairwise network mechanisms in the host signaling response to coxsackievirus B3 infection. Proceedings of the National Academy of Sciences, 107(39): 17053-17058.
36. Sean, P. and Semler, B. 2008. Coxsackievirus B RNA replication: Lessons from Poliovirus. Springer: 89-122.
37. Kuyumcu-Martinez,N., Van Eden, M., Younan, P., and Lloyd, R.E. 2004. Cleavage of poly(A)-binding protein by Poliovirus 3C protease inhibits host cell translation: a novel mechanism for host translation shutoff. Molecular Cell Biology, 24: 1779-1790.
38. Dorobantu, C.M., van der Schaar, H.M., Ford, L.A., Strating, J.R., Ulferts, R., Fang, Y., Belov, G. and van Kuppeveld, F.J. 2014. Recruitment of PI4KIIIβ to Coxsackievirus B3 replication organelles is independent of ACBD3, GBF1, and Arf1. J. Virol., 88: 2725-2736.
39. Hsu, N.Y., Ilnytska, O., Belov, G., Santiana, M., Chen, Y.H., Takvorian, P.M., Pau, C., van der Schaar, H., Kaushik-Basu, N., Balla, T. and Cameron, C.E. 2010. Viral Reorganization of the secretory pathway generates distinct organelles for RNA replication. Cell, 141: 799-811.
40. Melia, C.E., van der Schaar, H.M., Lyoo, H., Limpens, R.W., Feng, Q., Wahedi, M., Overheul, G.J., van Rij, R.P., Snijder, E.J., Koster, A.J. and Bárcena, M. 2017. Escaping host factor PI4KB inhibition: Enterovirus genomic RNA replication in the absence of replication organelles. Cell Reports, 21: 587-599.
41. Herold, J. and Andino, R. 2001. Poliovirus RNA replication requires genome circularization through a protein-protein bridge. Molecular Cell, 7: 581-591.
42. Barton, D., O’Donnell B., and Flanegan, J. 2001. 5’ cloverleaf in poliovirus RNA is a cis-acting replication element required for negative-strand synthesis. The EMBO Journal, 20: 1439-1448.
43. van Ooij, M.J., Vogt, D.A., Paul, A., Castro, C., Kuijpers, J., van Kuppeveld, F.J., Cameron, C.E., Wimmer, E., Andino, R. and Melchers, W.J. 2006. Structural and functional characterization of coxsackievirus B3 CRE(2C): role of CRE(2C) in negative- and positive-strand RNA synthesis. Journal of General Virology, 87: 103-113.
44. Brunner, J.E., Nguyen, J.H., Roehl, H.H., Ho, T.V., Swiderek, K.M. and Semler, B.L. 2005. Functional interaction of heterogeneous nuclear ribonucleoprotein C with Poliovirus RNA synthesis initiation complexes. J. Virol., 79: 3254-3266.
45. Vanerjee, R., Echeverri, A., and Dasgupta, A. 1997. Poliovirus-encoded 2C polypeptide specifically binds to the 3’-terminal sequences of viral negative-strand RNA. Journal of Virology. 71: 9570-9578
46. Feuer, R., Mena, I., Pagarigan, R.R., Hassett, D.E. and Whitton, J.L. 2004. Coxsackievirus replication and the cell cycle: a potential regulatory mechanism for viral persistence/latency. Medical Microbiology and Immunology, 193: 83-90.
47. Sin, J., Mangale, V., Thienphrapa, W., Gottlieb, R.A. and Feuer, R. 2015. Recent progress in understanding coxsackievirus replication, dissemination, and pathogenesis. Virology, 484: 288-304.
48. Chen, Y.H., Du, W., Hagemeijer, M.C., Takvorian, P.M., Pau, C., Cali, A., Brantner, C.A., Stempinski, E.S., Connelly, P.S., Ma, H.C. and Jiang, P. 2015. Phosphatidylserine vesicles enable efficient en-bloc transmission of Enteroviruses. Cell, 160: 619-630.
49. Robinson, S.M., Tsueng, G., Sin, J., Mangale, V., Rahawi, S., McIntyre, L.L., Williams, W., Kha, N., Cruz, C., Hancock, B.M. and Nguyen, D.P. 2014. Coxsackievirus B exits the host cell in shed microvesicles displaying autophagosomal markers. PLOS Pathogens, 10: e1004045.
50. Wong, J., Zhang, J., Si, X., Gao, G., Mao, I., McManus, B.M. and Luo, H. 2008. Autophagosome supports Coxsackievirus B3 replication in host cells. J. Virol., 82: 9143-9153.
51. Senior, K. 2002. FDA panel rejects common cold treatment. The Lancet Infectious Diseases. 2: 264.
52. Ma, J.D., Nafziger, A.N., Rhodes, G., Liu, S., Gartung, A.M. and Bertino, J.S. 2006. The effect of oral pleconaril on hepatic cytochrome P450 3A activity in healthy adults using intravenous midazolam as a probe. The Journal of Clinical Pharmacology, 46: 103-108.
53. Groarke, J. and Pevear, D. 1999. Attenuated virulence of Pleconaril-resistant coxsackievirus B3 variants. The Journal of Infections Diseases, 179: 1538-1541.
54. Makarov, V.A., Braun, H., Richter, M., Riabova, O.B., Kirchmair, J., Kazakova, E.S., Seidel, N., Wutzler, P. and Schmidtke, M. 2015. Pyrazolopyrimidines: Potent inhibitors targetiing the capsid of Rhino- and Enteroviruses. ChemMedChem., 10: 1629-1634.
55. Pinkert, S., Dieringer, B., Diedrich, S., Zeichhardt, H., Kurreck, J. and Fechner, H. 2016. Soluble coxsackie- and adenovirus receptor (sCAR-Fc); a highly efficient compound against laboratory and clinical strains of coxsackie-B-virus. Antiviral Research, 136: 1-8.
56. De Palma, A.M., Heggermont, W., Lanke, K., Coutard, B., Bergmann, M., Monforte, A.M., Canard, B., De Clercq, E., Chimirri, A., Pürstinger, G. and Rohayem, J. 2008. The thiazolobenzimidazole TBZE-029 inhibits enterovirus replication by targeting a short region immediately downstream from Motif C in the nonstructural protein 2C. J. Virol., 82: 4720-4730.
57. Hunziker, I.P., Harkins, S., Feuer, R., Cornell, C.T. and Whitton, J.L. 2004. Generation and analysis of an RNA vaccine that protects against coxsackievirus B3 challenge. Virology, 330: 196-208.
58. Kemball, C., Alirezaei, M., and Whitton, J. 2010. Type B coxsackieviruses and their interactions with the innate and adaptive immune systems. Future microbiology, 5(9): 1329-1347.
59. Fung, G., Luo, H., Qiu, Y., Yang, D. and McManus, B. 2016. Myocarditis. Circulation research, 118(3): 496-514.
60. Garmaroudi, F.S., Marchant, D., Hendry, R., Luo, H., Yang, D., Ye, X., Shi, J. and McManus, B.M. 2015. Coxsackievirus B3 replication and pathogenesis. Future microbiology, 10(4): 629-653.
61. Xiong, D., Yajima, T., Lim, B.K., Stenbit, A., Dublin, A., Dalton, N.D., Summers-Torres, D., Molkentin, J.D., Duplain, H., Wessely, R. and Chen, J. 2007. Inducible cardiac-restricted expression of enteroviral protease 2A is sufficient to induce dilated cardiomyopathy. Circulation, 115(1): 94-102.
62. Badorff, C., Lee, G.H., Lamphear, B.J., Martone, M.E., Campbell, K.P., Rhoads, R.E. and Knowlton, K.U. 1999. Enteroviral protease 2A cleaves dystrophin: evidence of cytoskeletal disruption in an acquired cardiomyopathy. Nature medicine, 5(3).
63. Wessely, R., Klingel, K., Santana, L.F., Dalton, N., Hongo, M., Lederer, W.J., Kandolf, R. and Knowlton, K.U. 1998. Transgenic expression of replication-restricted enteroviral genomes in heart muscle induces defective excitation-contraction coupling and dilated cardiomyopathy. Journal of Clinical Investigation, 102(7): 1444.
64. Alidjinou, E.K., Sane, F., Engelmann, I., Geenen, V. and Hober, D. 2014. Enterovirus persistence as a mechanism in the pathogenesis of type 1 diabetes. Discovery medicine, 18: 273-282.
65. Fechner, H., Pinkert, S., Geisler, A., Poller, W. and Kurreck, J. 2011. Pharmacological and biological antiviral therapeutics for cardiac coxsackievirus infections. Molecules, 16: 8475-8503.
66. Organtini, L.J., Makhov, A.M., Conway, J.F., Hafenstein, S. and Carson, S.D. 2014. Kinetic and Structural Analysis of Coxsackievirus B3 Receptor Interactions and Formation of the A-Particle. Journal of Virology 88(10): 5755–5765.
67. Tracy, S., Oberste, M.S. and Drescher, K.M. eds., 2008. Group B coxsackieviruses (Vol. 323). Springer Science & Business Media.