Polymer Degradation by Roseateles depolymerans
By Sean Smith
Introduction

Plastic waste in landfills and marine environments serves as one of the principle waste issues facing the world. As use of plastic materials continues over time, landfills will eventually not be able to hold the vast quantities of non-biodegradable plastics flooding their grounds. The development of biodegradable plastics as alternatives to the non-degradable products overwhelming landfills has spiked in an attempt to solve the plastics problem facing our world. Two classes of plastic polymers that have arisen as potential alternatives to non-biodegradable but widely used aromatic polymers are aliphatic polymers and aliphatic-aromatic co-polymers. Each of those two classes of plastics holds different physical and chemical properties that make them adept at filling a wide range of consumer needs from plastic grocery bag production to automobile manufacturing.
Both aliphatic polymers and aliphatic-aromatic co-polymers are biodegradable by a wide range of microbes. The mechanisms by which each species degrades these polymers differs and is dependent on the class of enzymes utilized by the bacteria. Furthermore, each microbial species has a limit on the different classes of polymers that it can biodegrade. This limit is known as substrate specificity.
Roseateles depolymerans is a well characterized species of microbe that has been shown to biodegrade a wide range of different polymers under mesophilic conditions. Therefore, R. depolymerans has been thoroughly studied for its potential use in landfills to in part solve the plastics problem. The following is a summary on the physical and chemical properties of aliphatic polymers and aliphatic-aromatic copolymers, the general mechanisms of microbial biodegradation of polymers, and a specific study on the properties of R. depolymerans and how those properties may prove useful for industrial applications of the species and enzymes produced by the species to landfills in an attempt to biodegrade environmentally harmful plastics into their last harmful constitutive parts.
Properties and Applications of Aliphatic and Aromatic Polymers
Aliphatic Polycarbonates and Polyesters are in many ways limited by their physical properties. Aliphatic Polycarbonates and Polyesters hold the environmentally friendly properties such that their biodegradation is relatively favored. Biodegradability is determined by three major categories of factors: The surface conditions of the plastic, which includes surface area, hydrophillic and hydrophobic properties, the first order structures, which includes molecular weight and chemical structure, and the high order structures, which includes crystal structure, elasticity and melting temperatures. In general, more flexible, loosely packed polymers with lower melting temperatures are more susceptible to biodegradation. Such chemical and physical properties are overwhelming common to both aliphatic polycarbonates and polyesters (16, 2).
As research on Aliphatic Polycarbonates and Polyesters has continued, it has been established that due to such poor mechanical and physical properties, which incidentally aid in ease of biodegradation, aliphatic polycarbonates will never entirely replace conventional plastics, however, despite a more limited scope of application, aliphatic polycarbonates and polyesters can still prove vital in some applications. Aliphatic polyesters have been marketed by a host of big market chemical companies including Dupont and Mitsubishi Chemical Co. and have been applied to the production of compost bags, packaging containers and transparent film for food wrapping. Those applications are not inhibited by the polycarbonate and polyester’s less than ideal resistance to high temperatures and capitalize on the compounds relatively impressive flexibility (16,8). Aromatic Polycarbonates and Polyesters have vastly different chemical and physical properties than their aliphatic counterparts. Research into the biodegradation of Aromatic Polymers has proven fruitless so far and an organism capable of effective biodegradation of such polymers has not been isolated. Such a phenomenon is not surprising when one investigates the physical properties of these polymers. Aromatic polymers have desirable impact resistance, high temperature resistance and retain ductility even under extreme conditions and high stress. When one examines Aromatic polymers under the criteria determining the biodegradability of a compound mentioned above, their lack of biodegradability can be explained as predicted. Their high temperature resistance makes the degradation endergonic and the ability to maintain shape under high stress lessens enzymatic ability to access bonds whose breakage is key to the degradation pathway (7,8).
Due to their durability and impressive temperature resistance, aromatic polymers have been applied to a wide range of industrial, medicinal, and commercial fields. Specifically, aromatic polymers have been used in construction of automobiles, medical catheters, syringes, and aircraft. They have also entered the hope as a key material for sporting goods, water dispensers, and photography equipment. However, the waste generated from aromatic polymers enters both landfills and marine environments as a solid contaminate that cannot be effectively biodegraded. The continued unregulated use of such plastics would continue to harm the environment in increasingly more gruesome ways and marine and landfill plastic waste increases in volume (4).
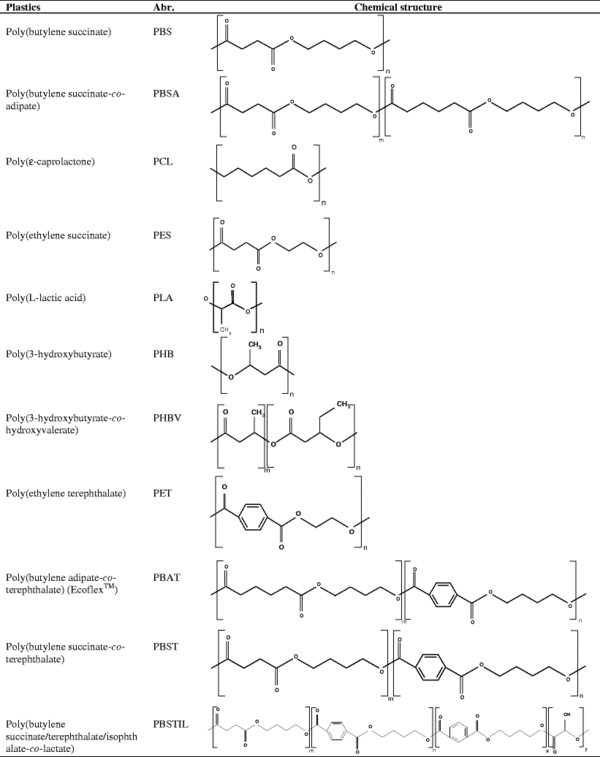
Preparation of Aliphatic Polymers
Aliphatic Polycarbonates and Polyesters has three different pathways of synthesis. They are either synthesized by phosgenation of hydroxyl compounds, transesterification of diols or through ring opening polymerization. These three methods have differing environmental impacts. Phosgenation of hydroxyl compounds is known for its high yield of product on an industrial scale but employs hazardous and toxic phosgenes and pyrimidines during synthesis and produces environmentally deleterious compounds as byproducts that much be meticulously disposed of through additional steps. Transesterification of diols with lower dialkyl carbonates requires the presence of a catalyst and often a specific enzyme catalyst is employed to ensure regiospecificity of the reaction. Toxic byproducts such as phenol have been cited as side products of this reaction. This pathway of synthesis has proven to be most adept at yielding high molecular weight polymers. Ring-opening polymerization is catalyzed by organometallic reagents. Such a method requires complex catalysts with great specificity, but has been shown to potentially have a less negative environmental impact in comparison to the other synthetic methods (5, 16, 7).
Importance and Applications of Aliphatic-Aromatic Polycarbonates and Polyesters
In an attempt to capture the durability and high temperature resistance of aromatic polymers while maintaining the biodegradability of aliphatic polymers, unique aliphatic-aromatic polycarbonates and polyesters have been synthesized, characterized, and applied to an amalgam of industries. Characterization of aliphatic-aromatic polymers revealed that, just as expected, these compounds retained both excellent mechanical properties sufficient to replace the use of non-biodegradable aromatic polymers and biodegradability. Therefore, while aliphatic polymers proved an incomplete answer to the issue of plastic waste overwhelming landfills, the directed synthesis of aliphatic-aromatic compounds may serve as the solution. Effective biodegradability of such polymers was only recently characterized by Shah et al. in 2013. Once the biodegradability of the polymers was established, the drive to find application of such polymers increased exponentially. It is hypothesized that such polymers could and should replace aromatic polymers in many industries (16, 1, 3).
General Mechanisms of Polymer Biodegradation
Biodegradation of polymers can occur through a whole host of forms outlined in Figure 3. The most common general form of polymer degradation exhibited by microbes involves the secretion of extracellular depolymerization enzymes onto the a polymer that is outside of the cell, but also proximate to the cell. The depolymerization enzymes shown in Figure 4 degrade the polymer into oligomers and then eventually into water soluble monomers. These monomers can then pass through the semipermeable cell membrane and be utilized as carbon or nitrogen sources by the bacterium (4).
There are two different types of polymer degradation in relation to the manner in which the polymer is attacked. There is surface erosion degradation, which includes the aforementioned extracellular enzyme mechanism and there is bulk erosion. While surface erosion only degrades the outer layer of the polymer, bulk erosion degrades the polymer from the inside and out and requires access to the internal structure of the compound undergoing degradation (4).
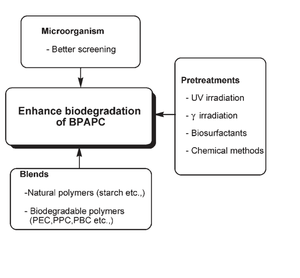
The two major biodegradation mechanisms are biological oxidation and hydrolysis. Hydrolysis, or hydrolytic degradation, is further segregated into two categories: catalytic hydrolysis and non-catalytic hydrolysis. Catalytic hydrolysis is the mechanism of extracellular depolymerization enzymes necessary to the depolymerization of aliphatic and aliphatic-aromatic polymers and copolymers since it requires the use of esterases, lipases and depolymerases. Conversely, non-catalytic hydrolysis relies on metals and acids naturally found in the soil to initiate polymer degradation. Biological oxidation is less applicable to aliphatic and aliphatic-aromatic copolymer breakdown and relies on enzymes of UV light to oxidize polymers and thus breakdown polymer chains into monomeric parts (4, 10).

Survey of Species with Polymer-Degradation Potential and a Survey of Polyester-Degrading Enzymes
While Roseateles depolymerans is one of the most well characterized species with polymer bio-degradation ability, is it one of many. While not all species exhibit the same impressive ability to biodegrade a wide array of different polymers, the total number of polymers that have been deemed biodegradable due to characterization of microbes is increasing. However, like Roseateles depolymerans these species are limited to the biodegradation of aliphatic polymers and aliphatic-aromatic copolymers. The following is a survey of a whole host of species exhibiting polymer biodegradation and a summary of the characterized enzymes responsible for the metabolism. Figure 5 summarizes the main categories of microbial enzymes responsible for the degradation of polymers, an example of species contain such an enzyme, and that enzyme’s substrate specificity. Each of these enzymes attacks a unique and specific part of the polymer (16, 12, 13).
Esterase enzymes degrade polymers by breaking down esters into alcohols and acids. Enzyme activity is reliant on the ability to access the ester for attack and enzymatic activity can be slowed if a tightly packed crystal structure depresses the accessibility of the ester site. The enzymes responsible for biodegradation activity in Roseateles depolymerans have been categorized as esterases and display a wide range of substrate specificity. Another species that has been shown to utilize esterase enzymes for biodegradation is Thermobifida alba. T. alba is a thermophilic bacterium that produces a thermostable esterase enzyme that has also been shown to degrade aliphatic-aromatic copolymers. Such a bacterium could prove useful in the event that biodegradation needed to occur at temperatures too high for effective biodegradation by R. depolymerans. However, T.alba displayed a very narrow range of substrate specificity in relation to R.depolymerans (16,9).
A second class of polymer biodegradation enzymes is cutinase. Cutinase enzymes have the ability to hydrolyze a wide variety of synthetic and organic esters and in addition to the ability to breakdown polymers, cutinase enzymes have also been shown to degrade long and short chain triglycerides. Of the four species mentioned in Figure 5, Pseudozyma antarctica had the widest range of substrate specificity and could effectively biodegrade PCL, PLA, PBS, and PBSA. P. antarctica showed an impressively affinity for polymer degradation in a study done by Shinozaki et al. However, it is an extremophile isolated originally from antarctica and the application of such a bacterium or its enzymes to polymer degradation under mesophilic conditions may prove difficult (11,14).
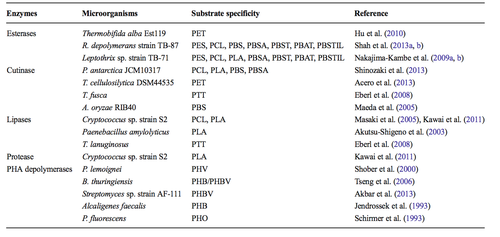
A third class of polymer biodegradation enzymes is lipase. Lipase enzymes are a subcategory of esterase enzymes and are known for their breakdown of fatty acids. Lipase enzymes have also been found to attack the ester bond of polymers and to degrade polymers through hydrolysis, but exhibit a unique catalytic site. This unique catalytic site causes a narrow range of substrate specificity. All of the species enumerated in Figure 5 can only biodegrade one or two specific polymers. Cryptococcus sp. utilizes lipase enzymes to biodegrade polymers and has been studied by Masaki et al. and Kawai et al. This species shows comparatively good variety in substrate specificity in comparison to other species reliant on lipase enzymes as it can degrade PCL and PLA. Cryptococcus is a relatively well characterized genus of fungus. It is important to note that biodegradation of polymers is not limited to bacteria and appears to have independently evolved in a variety of fungi as well (14).
The fourth and final major class of biodegradation enzymes is the PHA depolymerates. The mechanism of PHA depolymersases is such that a hydroxyl group must be present in the polymer to allow for effective biodegradation. Therefore, the range of substrate specificity for this class of enzymes is narrow. However, this class of enzymes, while attacking only a narrow group of substrates, has the ability to biodegrade a group of polymers not broken down by the remaining classified microbial enzymes with biodegradation activity. The most recently classified PHA depolymerates enzymes were isolated from Streptomyces sp. Strain AF-111 by Akbar et al. in 2013 (16,17,15).
Roseateles depolymerans Background and Discovery
Roseateles depolymerans was first isolated the Hanamuro River in Tsukubam Ibaraki Prefecture of Japan on a medium containing poly(hexamethylene carbonate) (PHC), a high molecular weight biodegradable polymer useful as both the building blocks for specialty polyurethanes and as more environmentally friendly commercially distributed plastic. While R. depolymerans was originally isolated due to its ability to biodegrade only PHC, its biodegradation abilities have proved diverse. R.depolymerans has not only been shown to degrade an amalgam of aliphatic polyesters including polybutylene succinate (PBS), polycaprolactone (PCL), and poly(butylene carbonate) (PBC), but also has been shown to breakdown more durable aliphatic-aromatic copolyesters such as poly(butylene succinate)-co-(butylene adipate) (PBSA). This diverse biodegradation ability could be applied to the field of waste management, specifically in biochemical monomer recycling, and could greatly aid in the industrial-scale breakdown of many durable, environmentally friendly plastics. Furthermore, the elucidation of the R. depolymerans biodegradation mechanism may open doors to the development of innovative, high-quality biodegradable plastics. R. depolymerans is one of the more well characterized microbes with polymer-degradation ability. However, the number of characterized microbes with the ability biodegrade both aliphatic polyesters and aliphatic-aromatic polyesters is always increasing (8, 6, 3).
Cell Structure and Metabolism
Individual Roseateles depolymerans cells are Gram-negative, flagellated and therefore motile, straight rods that grow optimally at pH 6.5 and at 35 °C. However, cells can survive between pH 5-8 and under standard mesophilic conditions. The bacterium is an aquatic obligate-aerobic β-subclass proteobacteria capable of producing bacteriochlorophyll (BChl) a and carotenoid pigments and is the only known aerobic phototrophic bacteria in that subclass. Even under conditions of high light cells cannot survive anaerobic conditions. Cells form polyhydroxybutyrate (PHB) granules as a reserve carbon and energy source. The bacterium can grow and reproduce through binary fission under heterotrophic conditions with mannitol, D-fructose, pyruvate, D-galactose, lactate, L-malate, succinate, D-glucose, citrate, Casamino acids, or yeast extract as the sole carbon source. In the presence of rich media the bacterium is weakly pigmented, but as carbon concentration decreases, the bacterium increases pigment production and becomes rose-pink in color. The bacterium is negative for nitrogen fixation but positive for gelatinase and oxidase. Most importantly R. depolymerans degrades aliphatic and aliphatic-aromatic polyesters through a partially elucidated co-metabolic system (3).
Isolation and Characterization of Roseateles depolymerans Esterase Enzymes
To date, only two enzymes produced by Roseateles depolymerans whose function has been connected to both aliphatic-aromatic co-polyester degradation and aliphatic polyester degradation have been isolated and characterized. These two enzymes, named Est-H and Est-L, have been categorized as a type of esterases. An esterase is a type of enzyme that degrades esters into an acid and an alcohol through hydrolysis. Est-H and Est-L were found to be 31 kDa and 27 kDa respectively (6).
Est-H and Est-L showed substrate specificity. While both Est-H and Est-L exhibit substrate specificity, their enzymatic activities relative to each other at each substrate are not significantly different. When each enzyme was exposed to p-nitrophentl acyl esters of carbon chain lengths between two and eighteen, enzymatic activity peaked at the six member carbon chain and decreased steadily as the chain shortened from six carbons to two and as the chain lengthened from six carbons to eighteen. Such a trend is common to esterase enzymes (16).
Est-H and Est-L both exhibit maximum enzymatic activity under mesophilic conditions and at neutral pH. Both enzymes exhibited activity between 20 and 45 °C and between pH 5.0 and 11.0. The optimum temperature for enzymatic activity was 30 °C and 100% of enzymatic activity was retained between pH 8.0 and 10.0. Activity at a wide range of pHs may prove to be useful in the eventual application of Est-H and Est-L as plastic biodegraders (6).
Est-H and Est-L display the ability to biodegrade an impressively wide array of aliphatic and aliphatic-aromatic polymers. To date, R. depolymerans has been shown to degrade PES, PCL, PBS, PBSA, PBST, PBAT and PBSTIL. Therefore, Est-H and Est-L have the properties to be applied to the environmentally friendly process of biochemical monomer recycling. As eco-friendly aliphatic polymers and aliphatic-aromatic polymers increase in prevalence Est-H and Est-L will become increasingly integral to the process of waste management in both landfills and potentially marine environments (6,16).
Regulation of Photosynthetic Apparatus
Roseateles depolymerans has been shown to contain a complex system of regulation for its photosynthetic apparatus that is reliant on the concentration of carbon surrounding the bacterium to determine the amount of photosynthetic pigments produced. However, to understand the regulation of the photosynthetic pathway, one must first understand the nuances of said pathway.

Roseateles depolymerans contains a reaction center (RC) where electron transfer occurs and a light-harvesting (LH) system comprised solely the LH1 complex typically bound to bacteriochlorophyll (BChl) a. Many like species contain both LH1 and LH2, but the presence of a single variety of complex is not uncommon. The building blocks of the LH1 complex and the RC are coded for by a single operon known at the puf operon. The accumulation of BChl a in the bacterium has been found to to correlate with the increased production of the photosynthetic apparatus. Accumulation of BChl a was found to increase when the cells were grown on a medium containing a low concentration of carbon sources. The regulation of the puf operon appears the be connected with decreased concentration of carbon sources and increased oxygen tension. Transcripts of the puf mRNA were only detected in cells under conditions favorable for the production of the photosynthetic apparatus.
However, Roseateles depolymerans only relies on its photosynthetic apparatus in limited conditions. It has been found that the bacterium does not utilize light as an aid for growth as the cells grow as statistically equivalent rates in both light and dark conditions. One condition where the bacterium has been found to rely on its photosynthetic apparatus is starvation. Under starvation conditions, utilization of energy from light may assist on the maintenance and upkeep of the cell and thus increase cell viability.
While the physiological significance of photosynthetic capabilities in alpha-proteobacteria has been well characterized, since R. depolymerans is the only obligate aerobic beta-proteobacterium with photosynthetic capabilities, its significance has been less fully investigated. However, the photosynthetic system has been linked as a potential integral factor to the polymer degradation pathway. The production and deployment of vital enzymes for polymer degradation has been connected to photosynthetic activities of the bacterium (18, 3).
Proposed Mechanism for Roseateles depolymerans Enzymatic Regulation and Activity
The proposed mechanism behind the regulation of Est-H and Est-L production links the activity of the Roseateles depolymerans photosynthetic apparatus to polymer biodegradation potential. Just as a decrease in oxygen concentrations had an effect on photosynthetic apparatus production, it also had an effect on enzyme secretion levels. As oxygen levels decreased, levels of enzymatic secretions increased. Shaa et al. hypothesized that since R. depolymerans is a unique organism in the sense that it contains BChl a but does not resemble autophototrophic or aerobic phototrophic bacteria that the photosynthesis of the strain much be in some way connected with the polymer biodegradation abilities, most specifically in production and excretion of enzymes. However, more research is needed on the subject before conclusions can be drawn (18, 16)
The proposed mechanism for enzymatic activity varies slightly with each polymer being degraded. However, the mechanisms can be generally grouped into aliphatic polymer degradation and aliphatic-aromatic copolymer degradation. Polybutylene Succinate-co-Adipate (PBSA) serves as a primary example of the bacterium’s mechanism for biodegradation of aliphatic polyesters. PBSA is degraded into its constitutive monomers, succinic acid, 1,4-butanediol and adipic acid. There are multiple proposed mechanisms for the accomplishment of that degradation. Each takes into account that succinic acid appears as a product of biodegradation before adipic acid. The first proposed mechanism is that depolymerization initiates as succinic acid segments. The depolymerization of the succinic acid segments forms a rumpling or a kink in the polymer crystal structure that allows enzymes to effectively degrade the adipic acid segments of the polymer chain. Each intermediate in the biodegradation of PBSA is outlined in Figure 7 and shows that PBSA is effectively degraded into a pentamer through attack of the succinic acid segments. The ability of esterase enzymes attack esters and form alcohols and acids is exhibited in each step of the degradation mechanism (16).

The mechanism for aliphatic-aromatic copolymer biodegradation by R. depolymerans is exemplified by the depolymerization of poly(butylene succinate/terephthalate/isophthalate)-co-(lactate) (PBSTIL). Each intermediate to the degradation pathway is outlined in Figure 8. Aliphatic-aromatic co-polymer depolymerization begins as aliphatic depolymerization does with the attack of succinic acid by the esterase enzyme leading to the generation of a host of long chain oligomers. The generation of pentamer intermediates has been shown to occur first, but the oligomers are continually attacked by the esterase enzyme until they have been full degraded into their monomeric parts (16,7).
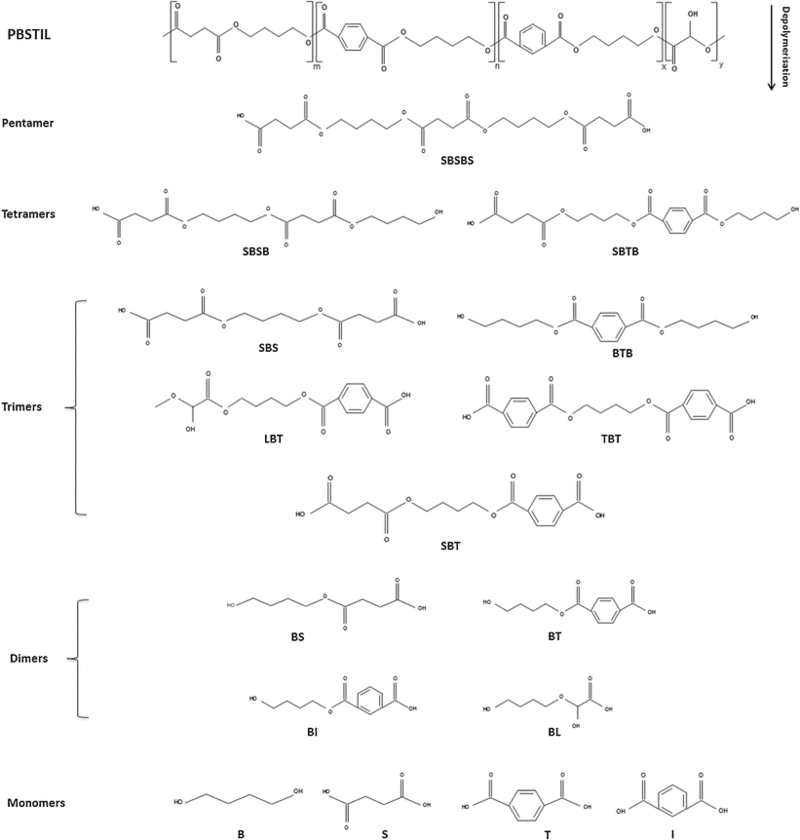
Widespread Applications of Polymer Biodegradation
As industrial producers move away from non-biodegradable aromatic polymers and move towards the most viable environmentally friendly alternative, aliphatic-aromatic co-polymers, R. depolymerans and species with the similar ability to biodegrade polymers will prove vital in solving the plastics problem in landfills. Species that survive under mesophilic conditions, such as R. depolymerans should prove most useful under most circumstances, due to the incredibly wide range of microbial diversity, species such as P. antarctica or T. alba could be applied to cold and hot situations respectively in the event that biodegradation of polymers was necessary in an extreme environment. The ability to isolate and reproduce the enzymes responsible to the biodegradation activity will greatly aid in solving the plastics problem, and as evidenced above, research is well on its way towards achieving in full that goal. Polymer degradation is a growing industry and as more and more biodegradable polymers replace environmentally hazardous non-biodegradable ones, it should continue to grow exponentially.
References
1) Pranamuda, Hardaning, and Rungsinma Chollakup. "Degradation of Polycarbonate by a Polyester-Degrading Strain, Amycolatopsis Sp. Strain HT-6." Applied and Environmental Microbiology 65.9 (1999): 4220. Web.
2) Suyama, Tetsuchi, Yutaka Tokiwa, and Pornpimol Ouichanpagdee. "Phylogenetic Affiliation of Soil Bacteria That Degrade Aliphatic Polyesters Available Commercially as Biodegradable Plastics." Applied and Environmental Microbiology 64.12 (1998): 5008-011. Web.
3) Suyama, Tetsuchi, Toru Shigematsu, and Shinichi Takaichi. "Roseateles Depolymerans Gen. Nov., Sp. Nov., a New Bacteriochorophyll A-containing Obligate Aerobe Belonging to the Beta-subclass of the Proteobacteria." International Journal of Systematic Bacteriology 49 (1999): 449-57. Web.
4) Artham, Trishul, and Mukesh Doble. "Biodegradation of Aliphatic and Aromatic Polycarbonates." Macromolecular Bioscience 8 (2008): 14-24. Web.
5) Jiang, Zhaozhong, Chen Liu, and Wenchun Xie. "Controlled Lipase-Catalyzed Synthesis of Poly(hexamethylene Carbonate)."Macromolecules 40 (2007): 7934-943. Web.
6) Shah, Aamer A., Tomoaki Eguchi, and Daisuke Mayumi. "Purification and Properties of a Novel Aliphatic-aromatic Co-polyesters Degrading Enzymes from Newly Isolated Roseateles Depolymerans Strain TB-87."Polymer Degradation and Stability 98 (2013): 609-18. Web.
7) Tokiwa, Yutaka, Buenaventurada P. Calabia, and Charles I. Ugwa. "Biodegradability of Plastics." International Journal of Molecular Sciences 10 (2009): 3722-742. Web.
8) Suyama, Tetsuchi, Hiroyuki Hosoya, and Yutaka Tokiwa. "Bacterial Isolates Degrading Aliphatic Polycarbonates." FEMS Microbiology Letters 161 (1998): 255-61. Web.8)
9) Hu, Xiaoping. "Diversity of Polyester-degrading Bacteria in Compost and Molecular Analysis of a Thermoactive Esterase from Thermobifida Alba AHK119." Applied Microbiology and 87 (2010): 771-79. Web.
10) Shaikh, Abbas-Alli. "Organic Carbonates." Chemical Reviews 96 (1996): 951-76. Web.
11) Longhi, Sonia, and Christian Cambillau. "Structure-activity of Cutinase, a Small Lipolytic Enzyme." Biochimica Et Biophysica Acta 1441 (1999): 185-96. Web.
12) Guebitz, Georg M., and Artur Cavaco-Paulo. "Enzymes Go Big: Surface Hydrolysis and Functionalisation of Synthetic Polymers." Trends in Biotechnology 26 (2007): 32-38. Web.
13) Shinozaki, Yukiko, Tomotake Morita, Xiao-hong Cao, and Shigenobu Yoshida. "Biodegradable Plastic-degrading Enzyme from Pseudozyma Antarctica: Cloning, Sequencing, and Characterization." Applied Microbiology and Biotechnology 97 (2013): n. pag. Web.
14) Masaki, Kazuo, Numbi R. Kamini, Hiroko Ikeda, and Haruyuki Iefuji. "Cutinase-Like Enzyme from the Yeast Cryptococcus Sp. Strain S-2 Hydrolyzes Polylactic Acid and Other Biodegradable Plastics." Applied and Environmental Microbiology 71 (2005): 7548-550. Web.
15) Gan, Zhihua, Qizhi Liang, Jie Zhang, and Xiabin Jing. "Enzymatic Degradation of Poly(e-caprolactone) Film in Phosphate Buffer Solution Containing Lipases." Polymer Degradation and Stubility 56 (1997): 209-13. Web.
16) Shah, Aamer A., Satoshi Kato, Noboru Shintani, and Numbi R. Kamini. "Microbial Degradation of Aliphatic and Aliphatic-Aromatic Co-Polymers." Applied Microbiology and Biotechnology 98 (2014): 3437-447. Web.
17) Akbar, Siddiq, Fariha Hasan, Akhtar Nadhman, Samiullah Khan, and Aamer Ali Shah. "Production and Purification of Poly(3-hydroxybutyrate-co-3-hydroxyvalerate) Degrading Enzyme from Streptomyces Sp. AF-111." Journal of Polymers and the Environment 21.4 (2013): 1109-116. Print.
18) Suyama, T., T. Shigematsu, T. Suzuki, Y. Tokiwa, T. Kanagawa, K. V. P. Nagashima, and S. Hanada. "Photosynthetic Apparatus in Roseateles Depolymerans 61A Is Transcriptionally Induced by Carbon Limitation." Applied and Environmental Microbiology 68.4 (2002): 1665-673. Print.