Engineered bacterial symbionts of Anopheles gambiae as inhibitors of Plasmodium falciparum
Introduction
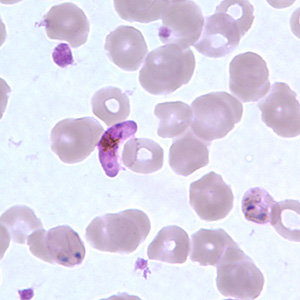
By Nontokozo Mdluli
Malaria is known to be the world’s deadliest parasitic disease, with over 800 million people contracting the disease annually. Malaria is the main cause of death in the tropical regions with at least 1 million death cases reported annually.[1] The disease is transmitted by vector mosquitoes such as the female Anopheles gambiae mosquito. The causative agent of malaria that is transferred from vector mosquitoes to hosts is the parasite Plasmodium, of the five known species of the parasite Plasmodium falciparum is the deadliest (Figure 1).[2]
In Africa, the mosquito Anopheles gambiae remains the most widely distributed Plasmodium falciparum vector in the region. Thus, malaria vaccines continue to be developed to find new mechanisms to control the disease. The current prescribed malaria vaccine involves immunization with an attenuated Plasmodium falciparum strain in its sporozoite form.[2] The initial stages of malaria vaccine development involved studies on Plasmodium berghei whose natural hosts are rodents. The rodents were immunized with X-ray irradiated, attenuated Plasmodium berghei sporozoites obtained from the salivary glands of infected mosquitoes.[2] Researchers discovered that immunized mice with the radiation attenuated parasite Plasmodium berghei developed immunity in later infections with the Plasmodium parasite. The development of immunity to later infections was an indication that a plausible target for inhibiting the complete transmission of the parasite Plasmodium in the mosquito vector had been discovered. The challenge since then has been developing a highly effective vaccine that directly targets the parasite in either the host cells or the mosquito vector.
In the past two decades, malaria vaccine development has expanded to target the three different stages of Plasmodium development. Vaccines have been developed to target the initial stage of mosquito vector to host transmission, which is the stage of liver cell infection. Other vaccines are being developed to target the erythrocytic stage of infection, whereby the host red blood cells are infected with the Plasmodium parasite. other vaccines being developed are directed to target the sexual stage, which is the phase at which the mosquito has taken up the parasite, and Plasmodium sexually reproduces midgut of the vector (Figure 2).[2] The most successful vaccine candidates to date have been the ones that target the initial phase of transmission of the parasite from mosquito vector to human host. The vaccine RTS,S has been the most developed and investigated vaccine candidate so far. The vaccine aims to target free circulating sporozoites in the host system, right before they invade the liver hepatocytic cells. The vaccine targets the Plasmodium CS protein which induces antibodies that respond to infection by sporozoites.[3] The RTS,S vaccine is currently in phase III testing in 5 -7 sub-Saharan African countries where malaria is endemic. Because the Plasmodium life cycle is so complex and because development of the parasite is so time sensitive, there have been a lot of challenges with developing the RTS,S vaccine, and many other vaccines.[2] Another combination of treatments aim to target the growth rate of the Plasmodium parasite in the sexual replication stage of development. Still, studies in malaria control continue to accelerate, focusing on better ways to deliver antigens in the mosquito vector in order to induce immunological responses to inhibit the parasite development in the mosquito vector.[3]
Research in malaria vaccine development recently expanded to look into more innate strategies of malaria control such as the host microbiota. Bacterial symbionts are abundant in a lot of organisms including insects that are disease vectors, such as mosquitoes and flies. Recent studies have tried to determine the mechanisms of these symbiotic relationships with the intentions of developing ways to intervene vector borne diseases such as malaria.[1] During transmission of sporozoites from infected host to vector mosquito, thousands of gametocytes may be ingested by the mosquito vector. These gametocytes later develop into gametes which fuse to produce zygotes. Successful fertilization of the gametes results to production of ookinetes. The ookinetes then proceed to invade and penetrate the epithelial cells lining the midgut of the mosquito vector where they develop into oocysts. When oocysts mature, they rupture releasing sporozoites which travel to the mosquito salivary glands waiting for the next transmission from the mosquito vector to a host.[3] The abundance of midgut microbiota in the mosquito vector creates a midgut bottleneck. With a large microbiota population colonizing the midgut of the mosquito, this compartment becomes a highly competitive environment for symbiotic bacteria as well as pathogenic bacteria.[1] Research has shown that the microbiota bottleneck present in the midgut of the mosquito vector has a severe impact on the development of Plasmodium oocysts, such that even if a mosquito vector ingests a large number of gametocytes, only a handful eventually develop into oocysts.[4] This interference in oocyst development in the midgut of the mosquito vector indicates that Plasmodium transmission success may be determined by microbiota composition;[4] if Plasmodium transmission can be effected by microbiota composition in the midgut of the mosquito vector, how can the mosquito midgut be genetically modified to express effector genes that inhibit Plasmodium development?[1] Paratransgenesis has been expanded in malaria vaccine development and treatment, by using genetically modified symbionts of the mosquito vector to produce effector molecules that interfere with Plasmodium development midgut of the mosquito vector.[5]
Plasmodium falciparum Life cycle
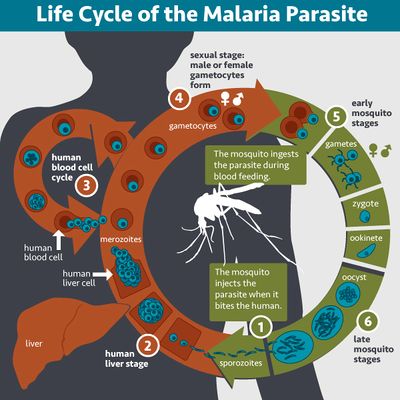
Plasmodium falciparum is a parasitic protozoa. It is one of five known Plasmodium species which cause the deadly malaria disease. The parasite is known to be transmitted from a mammalian host by female mosquito vectors. For human hosts, Anopheles gambiae is the primary transmitter of the Plasmodium falciparum parasite.[2]Plasmodium falciparum development occurs in two phases. In the first phase of development, the parasite reproduces asexually in the vertebrate host. In the second phase of development the parasite reproduces sexually in the mosquito vector.[2]
The first phase of development begins when infection is initiated by a female Anopheles gambiae mosquito biting into the vertebrate host (Figure 2). The Plasmodium falciparum parasite in the mosquito vector saliva enters the vertebrate host in the form of sporozoites. The sporozoites then proceed to invade the liver cells. After a few days of entering the host system, the sporozoites quickly infect the liver hepatocytes, reproducing asexually in the cells to form thousands of merozoites.[2] This stage of reproduction is known as the pre erythrocytic stage of the Plasmodium life cycle. The formation of merozoites in the mammalian host liver cells is followed by the erythrocytic stage, whereby the merozoites are dispersed into the host circulatory system from the liver, and invade the erythrocytes. At this stage, Plasmodium enlarges into a ring form called trophozoite, accompanied by ingestion of red blood cell cytoplasm. The trophozoites undergo multiple rounds of nuclear division resulting to the formation of schizonts. When the erythrocytes rupture, thousands of merozoites are released.[2] A few merozoite infected red blood cells will cease to reproduce asexually and the Plasmodium parasite will alternatively differentiate into mature sex gametocytes, which freely circulate in the bloodstream. Thus when a mosquito vector bites an infected vertebrate host, it ingests red blood cells filled with gametocytes. In the midgut of the mosquito vector, the red blood cells rupture, producing gametocytes which develop into gametes. The gametes then fuse to produce zygotes which eventually develop into ookinetes and proceed to invade and penetrate the epithelial cells in the gut of the mosquito vector. Once penetration is complete, the ookinetes develop into oocysts. The oocysts grow, mature, and undergo asexual replication to produce sporozoites. The sporozoites then travel to the mosquito vector’s salivary glands, completing the life cycle of the Plasmodium parasite, until the mosquito vector’s next blood transmission (Figure 2).[2]
Plasmodium falciparum development in the Anopheles gambiae midgut
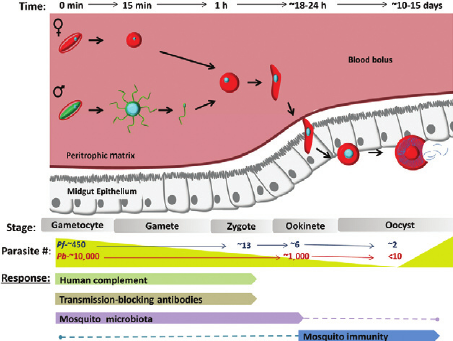
Plasmodium development is initiated when a mosquito vector initially ingests blood from an infected host. At the initial stage of infection, thousands of gametocytes are transferred into the circulatory system of the vector mosquito from the host. Not all of the ingested gametocytes survive the phases of development to mature into infectious parasites. The most dramatic losses in the Plasmodium parasite population is experienced when they reach very competitive regions in the mosquito vector[6](Figure 3). Studies estimate that of the thousands of gametocytes ingested by the mosquito vector, only 5 % develop into ookinetes, and only 10 % of those differentiate into oocysts.[7] In a study performed by Gouagna et al. (1998), Anopheles gambiae mosquitoes infected with Plasmodium falciparum from human hosts showed that of the 433.5 gametocytes detected from an infected human host, only 2.9 % formed trophozoites, 1.3 % formed ookinetes, and 0.8 8% formed oocysts in their respective, progressive stages of development.[7] This study suggests that the dramatic losses that the malaria parasite undergoes during its developmental cycle are due to different mechanisms, environmental and genetic, that act as initial responders to infections by the Plasmodium falciparum parasite in both the host and the mosquito vector.[7]
In order for a transmission cycle to be successful, gametocytes must enter the mosquito vector and differentiate into mature ookinetes, a process which can take up to 30 hours.[6] This process begins with a successful release of gametes from red blood cells ingested by the Plasmodium parasite from an infected host. Within an hour of being released from ruptured red blood cells, the gametes fuse to form a zygote. Successful fertilization is followed by an elongation of the zygote, a process which can take up to 10 hours, producing a slender ookinete.[6] The introduction of the Plasmodium falciparum parasite into the mosquito vector induces a series of enzymes which biochemically change the midgut of the mosquito vector. Studies show that some biological factors in the mosquito vector enable successful development of the Plasmodium ookinetes. Furthermore, some studies also show that some mosquito vectors have a mosquito gamete activation factor (GAF) which enhances the release of gametes from red blood cells ingested by the mosquito vector in vitro.[6] To determine the mechanism of the GAF factor Garcia, Wirtz & Rosenberg (1997) purified GAF from Anopheles stephensi; the GAF factor was transduced into Plasmodium falciparum and Plasmodium gallinaceum. The presence of the GAF factor induced exflagellation in P. falciparum and P. gallinaceum under conditions that would not normally enable the release of gametes of the parasite. The results of this study suggest that the surface of the gametes have GAF specific receptor sites present; it is also implied that a positive relationship exists between the development of the parasite and the mosquito vector.[6] Malaria vaccine development continues to target such relationships in order to control the spread of the disease.
The introduction of the Plasmodium parasite into the midgut of the mosquito vector also induces the release of enzymes that render the midgut of the mosquito a chemically and physically incompatible environment for the development of the Plasmodium falciparum parasite.[6] Some enzymes are secreted to catabolize the nutrients from the blood meal, which exposes the Plasmodium parasite to enzymes that may chemically degrade it in the process. Studies in mosquito midgut barriers show that adult female mosquitoes create a chitinous sack like barrier (PM) which surrounds blood ingested by the mosquito vector.[6] The PM is made of layers of chitin microfibrils on which other proteins, glycoproteins, and proteoglycans accumulate to form an envelope like structure which surrounds the entire food bolus containing Plasmodium gametocytes.[6] When the mosquito vector ingests blood from a host, the introduction of the food bolus into the midgut of the mosquito vector causes the midgut wall to swell, which induces the synthesis of PM,[6] which can be detected as early as after 2 hours of ingestion and desolated after the food meal is digested.[6] As an initial infection barrier, it has been suggested that the PM can be used as a mechanism for controlling the development of the parasite in the midgut of the mosquito vector. In a study by Billingsley and Rudin (1988) mosquitoes that were double fed with Plasmodium infected blood showed a thickened PM and a reduced oocyst cell count per gut. However, studies also show that the Plasmodium parasite in the form of ookinetes can secrete an enzyme called chitinase, which allows the ookinetes to penetrate the PM lining, resulting to a successful transmission cycle. Preliminary studies by Shahabuddin et al (1993) show that chitinase inhibition in mosquito vectors severely retards the development of ookinetes in the vector midgut.[6]
In addition to studying ookinete inhibition by innate immune system mechanisms such as the PM, studies have also looked at post ookinete penetration of the PM barrier and the implications of a successful transmission through survival of mature ookinetes. Once ookinete formation is completed, the ookinetes that survive the PM barrier rapidly penetrate the midgut epithelial cells, avoiding the degradative environment of the mosquito vector midgut lumen.[6] Some studies show that the ookinetes that manage to penetrate the PM barrier also develop resistance to the mosquito vector midgut enzymes; the parasites express protease resistant molecules on their surface as a protective mechanism from enzymes.[6] Successful ookinetes further develop into oocysts which eventually develop into sporozoites. The sporozoites will complete the transmission cycle by invading the mosquito vector salivary glands.[2] The pathway by which the ookinetes penetrate the midgut epithelial walls is not yet fully understood, studies suggest that several intracellular and intercellular pathways of penetration may exist for the parasite
Microbial Symbiosis of Anopheles gambiae
Microbial symbiosis plays an important role as an innate immune system barrier. Microbiota exist in different tissues within the organism, and at varying pH conditions. Their presence is tolerated by the immune system, as microbial presence confers some barrier to pathogens for the host. Furthermore, microbiota have been known to produce regulatory molecules which also act as a defense mechanism against pathogens that infect the host organism. These symbiotic microbes tend to out compete pathogenic microbes as a defense mechanism for the host, creating a bottleneck at crucial regions of pathogen development. In the mosquito vector, the midgut microbiota also plays a fundamental, beneficial role as an innate barrier to pathogens. They allow the mosquito vector to adapt to diverse environments by providing them with nutrients that they may not be able to obtain themselves, as well as facilitate species specific metabolism. Consider the distribution of mosquito species in different parts of the world, these insects are found in very humid conditions and sometimes very wet and hot conditions, and this adaptation may be attributed to the microbiota that exists within the mosquito vector, which influence the insect’s metabolism and choice of food. Therefore absence of these midgut microbiota might have very severe consequences on the metabolism of the mosquito vector, its growth rate, as well as its susceptibility to pathogens. Studies have shown that the presence of different microbes in the midgut of the mosquito vector can influence its interaction as a vector with the pathogen that infects it such as Plasmodium falciparum.[8]
The Anopheles gambiae mosquito is host to a large population of mutualistic symbiotic bacteria. Research shows that the most abundant microbes that occupy the Anopheles gambiae midgut are Asaia, Enterobacter, Mycobacterium, Sphingomonas, Serratia and Chryseobacterium.[8] When the Anopheles gambiae mosquito ingests blood infected with Plasmodium falciparum, the parasite is recognized as a foreign particle, which induces the response of the mosquito vector’s immune system. Studies have shown that Anopheles gambiae the midgut bottleneck created by the presence of symbiotic bacteria inhibit Plasmodium falciparum oocyst development.[8] In a study by Pumpuni, C.B. et al,[8] mosquito vectors that were fed blood infected with the Plasmodium parasite and with nonspecific symbiotic microbes showed partial or complete inhibition of oocyst development. In a different study by the same researchers, mosquito vectors fed blood infected with the Plasmodium parasite oocysts and nonspecific, non pathogenic symbiotic microbes had significantly lower oocyst development than groups of mosquito vectors that were not fed symbiotic microbes.[8] These results suggest that the midgut microbiota can inhibit Plasmodium development at the oocyst stage differentiation.[8] These microbes not only confer initial primary immunity to the presence of the Plasmodium parasite, however it has been suggested that they may potentially enhance the mosquito vector’s adaptive immune system. In one study, a group of mosquito vectors were fed blood infected with the Plasmodium parasite and foreign microbiota, and the control group was only fed infected blood without introduction of foreign microbes. After the initial encounter with the parasite, mosquito vectors with microbes present in their system tended to have a much stronger and effective response the second time that they are infected by the Plasmodium parasite than the control group.[8]
Symbiotic Control of Anopheles gambiae
Studies have shown that mosquito vector microbiota may play a very crucial role in controlling the spread of mosquito vector diseases such as malaria and African sleeping sickness.[5] The use of microbiota as an innate system to manage the development of Plasmodium falciparum in the mosquito vectors is currently being developed to enhance disease control. Research into two methods of using microbial symbionts as a strategy for disease control are currently under investigation. The first method considers the genetic modification of the microbial symbionts required by the mosquito vector. The symbionts of interest would be ones that exist in the midgut of the mosquito vector, and they would release regulatory molecules that suppress the target population. The second method considers the genetic modification of mosquito vector midgut microbes such that they affect the growth rate and vector capacity for parasite populations.[9]
In the past 10 years, research into genetic control methods has expanded to make targeting disease more selective and thorough. In order to use mosquito midgut symbionts as a control mechanism, genetically modified symbiotic bacteria are being developed to colonize the midgut of the mosquito vector. These bacteria have been modified to express molecules that can selectively regulate stages of development of the Plasmodium parasite and thus preventing successful transmission from the mosquito vector to a host.[5] This new strategy of genetic control is known as paratransgenesis. These genetic modifications to a few mosquito midgut microbes would potentially create a cascade of gene transfers to other populations. The techniques have not yet been used on a large scale and are currently under lab investigation.
Conclusion
Research in vaccine development has a lot of limitations because the treatments not only need to target the parasite of interest, but they need to only target the parasite and not consequently harm the host or by extension, disrupt ecosystems connected to the host. With promising results from various research with mosquito vector symbionts, further studies need to address the clear benefits of infecting mosquito vectors with microbes that do not naturally occur in those vectors, and what the potential genetic repercussions will be. For vaccines that are being developed to completely annihilate the Anopheles gambiae population, careful consideration must be taken about potential disruptions in the ecosystem; a lot of the studies on malaria vaccine development fail to mention any consequences of altering mosquitoes genetically, except to point out the benefits to the human population. In addition, further studies on effectively using innate systems such as the mosquito PM would potentially be a better alternative to combating the parasitic disease.
References
- ↑ 1.0 1.1 1.2 1.3 Sibao Wang, Anil K. Ghosh, Nicholas Bongio, Kevin A. Stebbings, David J. Lampe, and Marcelo Jacobs-Lorena (2012).Fighting malaria with engineered symbiotic bacteria from vector mosquitoes. Department of Molecular biology and Immunology, Malaria Research Institute, John Hopkins Bloomberg School of Public Health, Baltimore. PNAS July 31, 2012, vol. 109,no.31, pg 12734–12739.http://www.ncbi.nlm.nih.gov/pmc/articles/PMC3412027/pdf/pnas.201204158.pdf.
- ↑ 2.00 2.01 2.02 2.03 2.04 2.05 2.06 2.07 2.08 2.09 2.10 The History of Vaccines An Educational Resource by The College of Philadelphia.http://www.historyofvaccines.org/content/articles/malaria-and-malaria-vaccine-candidates Accessed 24 April 2016.
- ↑ 3.0 3.1 3.2 Jerome P. Vanderberg (2009). Reflections on Early Malaria Vaccine Studies, the First Successful Human Malaria Vaccination, and Beyond. HHS Public Access, National Institutes of Health (NIH) Public Access PA-manuscript. Vaccine. 2009 January 1; 27(1): 2–9. doi:10.1016/j.vaccine.2008.10.028. http://www.ncbi.nlm.nih.gov/pmc/articles/PMC2637529/
- ↑ 4.0 4.1 Boissie`re A, Tchioffo MT, Bachar D, Abate L, Marie A, et al. (2012) Midgut Microbiota of the Malaria Mosquito Vector Anopheles gambiae and Interactions with Plasmodium falciparum Infection. PLoS Pathog 8(5): e1002742. doi:10.1371/journal.ppat.1002742. http://journals.plos.org/plospathogens/article?id=10.1371/journal.ppat.1002742
- ↑ 5.0 5.1 5.2 Illiano V. Coutinho - Abreu, Kun Yan Zhu, and Marcelo Ramalho- Ortigao (2010). Transgenesis and Paratransgenesis to Control insect-borne diseases: Current Status and Future Challenges. National Institutes of Health PA-manuscripts, Parasitol Int. 2010 March ; 59(1): 1–8. doi:10.1016/j.parint.2009.10.002. http://www.ncbi.nlm.nih.gov/pmc/articles/PMC2824031/ Accessed 23 April 2016.
- ↑ 6.00 6.01 6.02 6.03 6.04 6.05 6.06 6.07 6.08 6.09 6.10 6.11 6.12 M. Shahabuddin (1998). Plasmodium ookinete development in the mosquito midgut: a case of reciprocal manipulation. Medical Entomology Section, Laboratory of Parasitic Diseases, National Institute of Allergy and Infectious Diseases, National Institutes of Health, Building 4, Room B2-37, Bethesda, Maryland 20892-0425, USA. Parasitology. 1998;116 Suppl:S83-93. http://journals.cambridge.org/download.php?file=%2FPAR%2FPAR116_S1%2FS0031182000084973a.pdf&code=a8c75a7c4ca5891f3d961c531b3262e2 Accessed 23 April 2016
- ↑ 7.0 7.1 7.2 Ryan C Smith, Joel Vega- Rodriguez, Marcelo Jacobs-Lorena (2011). The Plasmodium bottleneck: malaria parasite losses in the mosquito vector. Department of Molecular Microbiology, Malaria Research Institute, Johns Hopkins Bloomberg School of Public Health and Immunology, Baltimore, MD. National Institues of Health PA-manuscripts, Trends Parasitol. 2011 November ; 27(11): 514–522. doi:10.1016/j.pt.2011.05.001. http://www.researchgate.net/publication/262019195_The_Plasmodium_bottleneck_Malaria_parasite_losses_in_the_mosquito_vector.
- ↑ 8.0 8.1 8.2 8.3 8.4 8.5 8.6 Brian Weiss and Serap Aksoy (2011). Microbiome Influences on Insect Host Vector Competence. Department of Epidemiology and Public Health, Yale School of Public Health, New Haven, CT 06520, USA.Trends Parasitol. Author manuscript; available in PMC 2012 Nov 1.Trends Parasitol. 2011 Nov; 27(11): 514–522. http://www.ncbi.nlm.nih.gov/pmc/articles/PMC3179784/
- ↑ Irene Ricci, Matteo Valzano, Ulisse Ulissi, Sara Epis, Alessia Cappelli,and Guido Favia (2012). Symbiotic Control of Mosquito Borne Disease. School of Biosciences and Biotechnology, University of Camerino, Italy. Correspondence to: G Favia, School of Biosciences and Biotechnology, University of Camerino, via Gentile III da Varano, 62032 Camerino, Italy. Pathog Glob Health. 2012 Nov; 106(7): 380–385. http://www.ncbi.nlm.nih.gov/pmc/articles/PMC4001619/