Magnetotactic bacteria and their application
By Grant Miner
Overview
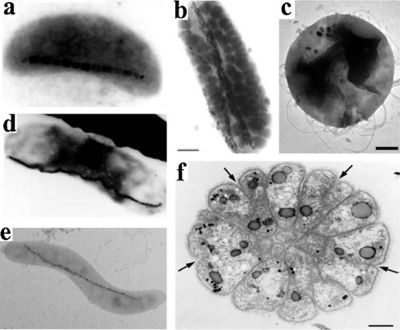
Magnetotactic bacteria (MTB) are a polyphyletic group of Gram-negative prokaryotes. Members of this group are characterized by their ability to align themselves and swim along a magnetic field. They owe this ability to the magnetosome, a specific intracellular structure that contains crystals of of magnetic metals (usually magnetite or greigite) bounded by a membrane. They were discovered in 1975 by Richard Blakemore. In his paper “Magnetotactic Bacteria” (Blakemore coined the name), he observed bacteria migrating along geomagnetic lines and, upon investigation, the magnetosome. This discovery impacted the scientific communities across many disciplines, including geology, mineralogy, chemistry, biochemistry, physics, oceanography, and, of course, microbiology. Because all species of MTB require microaerobic conditions, few species have been cultivated in pure condition. Because of this, they are relatively difficult to use in biotechnology. Nevertheless, researchers have succeeded in making headway in utilizing these strange organisms [1].
Physiology and Ecology
MTB usually inhabit sediments and water columns with vertical chemical stratification. Like many, bacteria, MTB’s main mode of taxis is through their helical flagella. Magnetotaxis causes these bacteria to orient themselves northward along geographic lines while in the northern hemisphere, and southward while in the southern. It is not completely known why these bacteria do this, but it is thought that heading in the direction of the nearest magnetic pole helps them reach areas of optimal oxygen concentration. Interestingly enough, MTB are observed to swim both directions while along the equator. [1] As a general rule, MTB are anaerobes, microaerophiles or facultatively anaerobic microaerophiles. Because of this, they are most likely to found at the the borders of oxic-anoxic regions as well as the anoxic regions of their habitat. In terms of a more physical placement within their habitat, MTB are most likely to be found at the water-sediment interface or thereabouts. [2]
A number of morphological types can be found within the MTB group including cocci, spirilla, vibrios and multicellular forms. MTB biomineralized two groups of metals: iron oxides and iron sulfides. Those species that produce iron sulfides biomineralized greigite (Fe3S4), while those that produce iron oxides biomineralized magnetite (Fe3O4). Additionally, species have been found that produce both and have magnetosomes that contain both magnetite and greigite. [1]
Geographic distribution of MTB’s are as widely varied as their morphology. As of today, only oxide-producers have been found in freshwater environments, which range from North America to Asia. MTB are even found within more extreme environments, such as lake Mono in California, an alkaline and saline in which the low solubility of iron makes the survival of MTB very difficult. Additionally, MTB are commonly found within the anoxic layers of marine environments, usually near the sedimentary layer. [2]
The Magnetosome
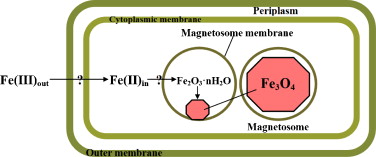
The magnetosome, the most important and defining part of the MTB, is an intracellular, membrane-surrounded magnetic iron inorganic crystal. Since its discovery, researchers have recognized it as a true prokaryotic organelle because of its complexity that rivals that of eukaryotic organelles. Despite its universal presence within MTB, the size, composition and morphology of the magnetosome varies between species. Usually, the size of the magnetosome ranges from 25mm-125mm, although 250nm magnetosomes have been observed in uncultured freshwater cocci. At normal temperatures, magnetosomes are permanently magnetic and form stable single-magnetic domains (meaning that they are uniformly magnetic). However, beyond normal temperatures and size ranges, magnetosomes are either unreliably magnetic or form multiple fields at smaller and larger than average temperatures, respectively. In terms of morphology, a few different forms of magnetosomes have been observed across species by various forms of electron microscopy.[1] These include, rectangular, bullet-shaped, octahedral and elongated prismatic morphologies. In most strains of MTB, the magnetosomes are fixed in several chains that are in turn attached to the cell in order to limit magnetostatic energy. The most commonly used minerals in the magnetosomes are, as mentioned previously, magnetite or greigite. However, many other iron sulfides have also been found within sulfide producing MTBS, such as cubic FeS and tetragonal FeS. The membrane that surrounds the crystal acts as a gate for pH, compositional and redox differentiation between the cellular environment and the vesicle. Its composition is thought be made up primarily of lipids, proteins, fatty acids, glycolipids, phospholipids and sulfolipids. [2]
The current model for Magnetosome formation consists of a few different processes:: vesicle formation, extracellular iron intake, iron transport into the magnetosome, and mineralization—although this knowledge mainly comes from the Magnetospirillum species, which is more readily culturable than other MTB. While it is known through direct observation (as well as common sense) that the later three steps are ordered temporally, it is unknown if vesicle formation comes before the uptake of iron or not. The most recent proposed model by Arakaki et al. (2008) lists three discrete steps. The first step consists of the creation of magnetosome membrane invaginations out of the cytoplasmic membrane (primed by GTPase), as well as the lining up of these vecicles along cytoskeletal filaments in order to form a chain.. Second, iron is transported into the cell. This transport is strictly regulated by an oxidation-reduction system because of the potentialy dangerous effects of having too much intracellular iron. In the third and final step, magnetosome proteins regulate morphology and trigger magnetite crystal nucleation. [1]
Cultivation and Magnetosome Isolation
Despite having an oxygen dependent respiratory metabolism, MTB cannot tolerate high levels of oxygen, as magnetosome formation require oxygen concentration between 1%-3%. Because of this, MTB are very difficult to culture on a large scale. The first bacteria to be isolated in pure culture was M. magnetotacticum. It’s thought that a possible cause for this oxygen sensitivity is a deficient amount of the oxygen protective enzyme catalase. Adding catalase to the growth medium has been shown to increase oxygen resistance. Additionally, M. gryphiswaldense and M. AMB-1 have equally high sensitivities to air. If cultured from large inocula, cultures generally show more of a resistance to atmospheric air. Once a large enough culture of bacteria has been grown, magnetosomes can be isolated through magnetic separation, sucrose gradient centrifugation, or combination of the two. After the membrane is dissolved by a detergent, the crystals tend to group together because of the attractive magnetic forces.
A readily available magnetic nanoparticle would be of great use to the biotech community. While such particles can be synthesized by various means, the processes available result in minerals that are not uniform in structure or magnetism. MTB, however, can supply a steady, standardized stream of uniform magnetic crystals. Additionally, biomineralization allows for the synthesis of these particles without needing to create any of the pH, temperature and pressure conditions usually needed for such synthesis. However, MTB application is not solely limited to the use of magnetosomes. This, MTB application can be fitted into two groups: full MTB and magnetosome-only biotechnology.
Live Magnetotactic Bacteria Application

One of the simplest applications for live MTB lies in their ability to detect magnetic fields in substances. In Funaki et. al 1992, MTB were applied to a surface of magnetite-rich pyroxenite. These bacteria were enriched by the S pole of a small hand magnet and were dropped onto the sample in a simulated geomagnetic field. Based on their habitat, these specific MTB were identified as north-seeking bacteria (which, confusingly enough, means that they will seek S poles). Ideally these bacteria would congregate around the S pole side of the drop in concordance with the simulated geomagnetic field. However, small clusters of these bacteria were found around the pockets of magnetite within the pyroxenite. These results suggest that MTB are more useful beyond determining whether or not a substance is magnetic or not. They can also, apparently, be used to determine the fine magnetic structures within a larger superstructure.[3]
Similarly, in Harasko et al. 1995, the same MTB cocci as well as M. Gryphiswaldense spirillum were evaluated for their abilities as a nondestructive method of domain analysis in soft magnetic metals. By applying these bacteria to a sample surface of SiFe in a manner similar to Funaki, researchers attempted to resolve domains in a sort of biological viewer. The intensity of the magnetic stray field-coated SiFe sheets were high enough to visualize the main domain structures even from a distance of 500 micrometers away. Because of the Brownian motion of live MTB bacteria, clearer pictures can be reached using larger domain sizes. However, dead MTB were found to be useful in visualizing smaller domains. This experiment proved the usefulness of MTB in creating relatively robust visualizations of magnetic domains. [4]
MTB are also useful in aquatic environments. In Rhoads 1995, researchers devised a “microbial magnetometer” for predicting oxygen depletion in bottom sediments and overlaying water—something that is based on microaerophilic and magnetotactic behavior in populations of magnetotactic bacteria. These bacteria were tracked using a magnetic sensor that determined their magnetic strength and position. [2]
A particularly extensive system of wastewater reclamation was outlined in Bahaj et al. 1998. Using previous observations of general tend of MTB to accumulate around local magnetic fields in a process called orientation magnetic separation (OMS), researchers hypothesized that these organisms could be used to reclaim water heavy in metal pollutants. Contaminated water would be run into a glass culture chamber containing MTB. These MTB would in turn bind to the metals and carry them down a line into a separator. Normally, in the Northern hemisphere, MTB orient themselves northwards along geomagnetic lines. However, some MTB orient themselves in the opposite direction. In order to ensure minimal levels of pollutants, this theoretical separator would have to extract all of these different kinds of bacteria. To accomplish this, North seeking bacteria that enter the separator on the north pole side are required to swim across the entire separator in order to reach the proper pole. This specific case was used to extract radionuclide Pu. The resulting bioaccumulation by MTB and the use of OMS has the capacity to be useful in removing radionuclides from waste water systems. Both of these methods of MTB application are facilitated by the fact that MTB, due to their magnetic nature, are easily counted and recorded by a magnetic recording head. This means that rough ideas of population numbers and concentrations are easily identifiable without going to great lengths [5]
In addition to their uses in aquatic environments, MTB also have many potential applications in the field of nanorobotics. While many breakthroughs in the field of nanorobotics have been made, a large barrier still remains in the form of power and control modules. These important pieces of machinery have historically proven particularly difficult to reduce to sufficient size to be useful in nanorobotics. One solution, however, is the use of cells that already have predeveloped power and control functions. In Martel et al. 2008, researchers hypothesized that flagellated nanomotors and nanometer-sized magnetosomes could act as an integrated steering and power system that would propel nanorobots to areas of the cell only reachable through small capillaries. In determining which strains to use, researchers determined that the most important characteristic to take into account was swimming speed. In light of this, they discounted spirillum, which tend to be slower, and decided to cocci, specifically the MC-1 cells. Researchers determined that the easiest method of bacterial control would be to simply create a field whose pole rested at the target position. In order to successfully control these bacteria, it was determined through experimental trial that a magnetic field of little over 0.5 gauss (the magnetic force of the earth) would be the least amount required to claim meaningful control over the cells. The selected cocci are the fastest of the magnetospirillum group, reaching speeds in excess of 300 m s1 h. In order to see these bacteria, researchers determined that the best and easiest method to detect the MTB while out on job was to use an MRI machine. Each magnetosome, despite being small, creates a disturbance in the magnetic field that is inside the scope of an MRI machine. The small, yet detectable, nature of the magnetosome (and the cells containing it) make MTB the ideal structures for controlled, measurable mobility while inside a human being. Researches have proposed four methods of moving synthetic objects. The first consists of fitting the particle within the MTB,. The second consists of loading the MTB with many small synthetic particles. The third consists of one MTB pushing a large particle from behind. The fourth, which is more useful for large particles, involves a swarm of MTB banding together to move one larger object. For applications requiring transport through capillary sections, say, to a tumor site, methods 1 is favored. This hypothesis was confirmed by researchers who successfully loaded and delivered fluorescent cell penetrating proteins across a vascular system to a target. For situations with multiple targets, method 2 is favored. This hypothesis was confirmed in another experiment, wherein biodegradable nanoparticles were attached to an MTB via antibodies, guided to a specific region and dispersed. [6]
Magnetosome Application
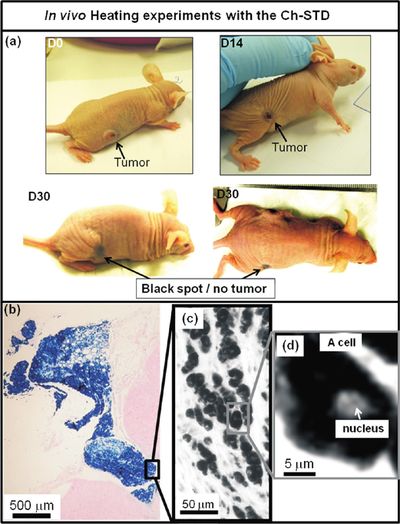
Magnetosomes are unique particles in that they are relatively standardized in composition, size and magnetism. Additionally, they come with their own membranes, making them ideal particles for a variety of biotech applications. One of the most common methods of magnetosome application is particle immobilization. Some of the first research in this field was done by Matsunaga and Kamiya 1987, where they limited the functions of enzymes glucose oxidase and uricase by immobilizing them crystals of synthetic magnetite harvested from magnetosomes. Really, “immobilization” is a misnomer, as the real object of this immobilization is actually isolation and labeling with magnetosomes in order to control the substances. A particular focus of these studies has been the extraction of DNA. Papers have remarked that, since phenol and chloroform are hazardous to human health, researchers need a new way to extract genomic DNA that they can perform regularly that isn’t as dangerous. A possible solution lies in the use of modified magnetosomes.[7] In Nakagawa et al. 2006, researchers attempted to extract genomic DNA through the use of magnetosomes (there called bacterial magnetic particles, or BMP’s) that were modified with 3-[2-(2-aminoethylamino)-ethylamino]-propyltrimethoxysilane (AEEA) to produce a dense amine surface. These modified magnetosomes were able to engage in electrostatic capture of DNA. The greatest recorded capture was 600 ng of DNA, which was captured with 10 μg of of aminosilane-modified magnetosomes. Additionally, these results were was enhanced when researches incubated the reaction at 80%, with DNA recovery from the magnetosomes approaching 95%. the mechanisms of this DNA recovery is as follows. The modified magnetosomes capture passively through magnetic attraction. After 20 mM phosphate buffer is added and the bath is incubated at 80 C, the DNA is released from the the magnetosomes and undergoes PCR amplification with a 5’ end biotin modified primer. These strands are then recovered via an SA-immobilized magnetosome, and the resulting DNA-magnetosome complex is denatured to one strand. AFter this, the strand is hybridized with a fluorescently-labeled oligo probe. The final step in the in the process is to magnetically separate the magnetosome-DNA complexes from the hybridized fluorescent strands. This is done by a magnet which is situated at the bottom of the well. This method provides a relatively efficient method of DNA extraction. In comparison with a previous experiment where the magnetic agent were aminosilane-coated microchips, the method of DNA recovery was 45%. The researchers hypothesized that these results had something to do with the nature of magnetosomes, although the specific interaction is as of yet unclear, and DNA release was nowhere near perfect. Unfortunately, further tests showed that DNA extraction in human blood was lower than expected. The initial amount of DNA used was estimated to be 165 ng, and the released DNA was estimated at 100 ng. This suggests that proteins within blood are being attracted to the modified magnetosomes before they’re able to take up DNA. [8]
Another potential application for isolated magnetosomes are their various novel interactions with human physiology. Specifically, researchers have been focusing on the antitumoral applications of magnetosomes. In Alphandery et al. 2011, researchers examined the ability of magnetosomes extracted from AMB-1 MTB in heat-killing tumor therapy. To that end, a suspension containing 1 mg of chained together magnetosomes was injected into a tumors made up of MDA-MB-231 human breast cancer cells xenografted onto a mouse. These tumors were then exposed to 183 kHz alternative magnetic fields and field strengths of 20, 40 and 60 mT. These magnetic currents would cause the magnetosomes to heat up and, consequently, destroy the cells into which they have diffused. Up to 100% of these cells were destroyed in some trials. In order to test whether it was specifically the magnetosome or just the sheer force of magnetic heating that was destroying these cells, the chain magnetosomes were tested against two different types of chemically synthesized superparamagnetic iron oxide nanoparticles currently being tested for the same effect, as well as dead whole MTB and individual magnetosomes. The tumor decreases of the mice were measured in normalized volume. During the 30 days following the initial treatment, group who was inoculated with chain magnetosome contained three mice with largely decreased tumor sizes and one mouse with no tumor at all. In contrast to the chain magnetosomes, there was no or little antitumoral activity observed in cases where mice were treated with identical suspensions of PEG molecules or by superparamagnetic iron oxide nanoparticles coated by citrate ions, which are (or rather, have been) tested for cancer therapy. These failures were hypothesized to be due to the fact that with those nanoparticles, the suspensions had a large amount of free iron, which has been known to cause oxidative stress to cells. While this might have resulted in cell death around the point of inoculation, this zone spread and damaged other areas. The researchers attributed the these results to three main reasons. The first was the large amount of heat which magnetosomes produce when they are exposed to alternative magnetic fields, a reaction that comes from their strongly magnetic nature. The second is their ability to magnetize the breast cancer cells. This enables efficient heating and cell death. The third and final reason is the arrangement of the magnetosomes in a chain, rather than isolated magnetosomes. This arrangement favours homogenous temperature distribution within the tumor issue. [9]
Conclusion
The application of magnetotactic bacteria can be simplified into two methodologies: those that use a live MTB, and those that use only the magnetosome. We primarily use the former when the application requires an active, self sustaining individual. For example, magnetosomes, size notwithstanding, could not hope to function as nanoparticle carrier. What makes the MTB special in these applications is its ability to move itself with a force stronger than that of magnetism. To draw all magnetic particles to a single point requires an especially strong force. To draw all MTB requires a force not much stronger than that of the natural magnetic field. Essentially, an MTB is bacteria that we can give orders to with a harmless, easily-generated force. In contrast, the magnetosome is used in applications where having a live bacteria would actually inhibit the process. The presence of MTB in a tumor would only needlessly complicate the process of heat-kill therapy and only serve to reduce the total amount of magnetic material. In this situation and many others, having a small, uniform magnetic particle is preferable to a relatively large bacteria. Unfortunately, many of these applications are still in an early stage. Perhaps in a few more years time, research will advance to a point where these fascinating little bacteria bacteria are at the forefront of biotechnology.
References
- ↑ 1.0 1.1 1.2 1.3 1.4 http://www.sciencedirect.com/science/article/pii/S094450131200047X Yan.. Magnetotactic Bacteria, Magnetosomes and their Application. Microbiological Research. 2012;167:507-19.]
- ↑ 2.0 2.1 2.2 2.3 http://link.springer.com/article/10.1007/s002530051547 Schuler.. Bacterial magnetosomes: microbiology, biomineralization and biotechnological applications. Applied Microbiology and Biotechnology.1999;52(4):464-73.]
- ↑ http://www.sciencedirect.com/science/article/pii/003192019290192X Funaki.. The S pole distribution on magnetic grains in pyroxenite determined by magnetotactic bacteria. Physics of the Earth and Planetary Interiors. 1992;70(3-4):253-60.]
- ↑ http://ieeexplore.ieee.org/xpl/freeabs_all.jsp?arnumber=364766&abstractAccess=no&userType=inst Harasko.. Domain analysis by means of magnetotactic bacteria. IEEE Transactions on Magnetics. 2002;31(2):938-49.]
- ↑ http://www.sciencedirect.com/science/article/pii/S030488539701130X Bahaj.. Continuous radionuclide recovery from wastewater using magnetotactic bacteria. Journal of Magnetism and Magnetic Materials. 1998;184(2):241-44).]
- ↑ http://0-journals.ohiolink.edu.dewey2.library.denison.edu/ejc/pdf.cgi/Martel_Sylvain.pdf?issn=02783649&issue=v28i0009&article=1169_mmnpftftiihc Martel.. MRI-based Medical Nanorobotic Platform for the Control of Magnetic Nanoparticles and Flagellated Bacteria for Target Interventions in Human Capillaries. The International Journal of Robotoics Research. 2009;28(9):1169-82.]
- ↑ http://link.springer.com/article/10.1007%2FBF00256663 Masunaga and Kamiya. Use of magnetic particles isolated from magnetotactic bacteria for enzyme immobilization. Applied Microbiology and Biotechnology. 1987;26(4):328-32.]
- ↑ http://onlinelibrary.wiley.com/doi/10.1002/bit.20904/full Nakagawa..Capture and release of DNA using aminosilane-modified bacterial magnetic particles for automated detection system of single nucleotide polymorphisms. Biotechnology and Bioengineering. 2006; 94(5):862-868.]
- ↑ http://ijr.sagepub.com/content/28/4/571.short Martel.. Flagellated Magnetotactic Bacteria as Controlled MRI-trackable Propulsion and Steering Systems for Medical Nanorobots Operating in the Human Microvasculature. International Journal of Robotics Research. 2009;28(4):571-82.]
Authored for BIOL 238 Microbiology, taught by Joan Slonczewski, 2016, Kenyon College.