Vibrio cholerae O1 Biotype El Tor: Virulence Factors
Vibrio cholerae O1 biovar El Tor

Vibrio cholerae (V. cholerae) is a gram-negative pathogenic bacterium that infects the small intestine. Symptoms include severe diarrhea, vomiting, and dehydration, which can be life-threatening if not treated promptly. There are more than 200 serogroups of V.cholerae, but only two – O1 and O139 – are associated with cholera epidemics.[1]
V. cholerae is highly motile, with a single polar flagellum that enables it to move quickly in liquid environments. It circulates between the aquatic environment and the human gut. Direct human-to-human transmission of cholera is rare. Instead, the bacteria survive, proliferate, and transmit via contaminated reservoirs, including rivers, ponds, coastal waters, and wells.[1]
Vibrio cholerae serogroup O1 biotype El Tor (V. cholerae O1 El Tor) was the dominant strain of the 7th global cholera pandemic. It possesses two circular chromosomes that encode for 3,885 total open reading frames (2,770 on Chr 1 and 1,115 on Chr 2). [2] Most essential cell function and pathogenicity genes – including those for DNA replication and toxin production – are on Chr 1. [2] The ability of V. cholerae to cause illness requires the production of several virulence factors, including the cholera toxin, hemolysin toxin, hemagglutinin protease, and toxin-coregulated pilus.
Virulence factors — or pathogenicity factors — are structures, molecules, and regulatory systems that enable pathogens to colonize hosts and counter their immune responses [3]. To initiate infection, V.cholerae must traverse the stomach acid and survive among bile and antimicrobial peptides in the small intestines [2]. El Tor strains have as many as 524 virulence-associated genes. Host immune and biological factors induce several of these survival, colonization, and pathogenicity genes [2] .
Cholerae Toxin
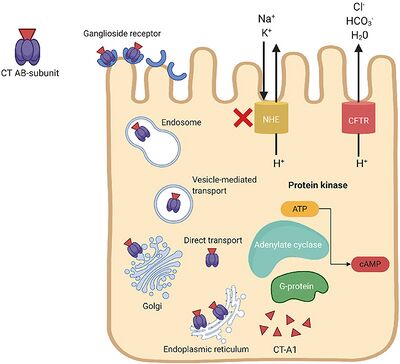
V. cholerae pathogenesis is attributed primarily to the cholera toxin (CT). It is coded for by genes on the core region of the CTXϕ prophage. These genes include ctxA and ctxB, which encode A and B subunits. [1] The cholera toxin CT consists of an A subunit (CTA) — responsible for enzymatic activity — and five B subunits (CTB). Once V. cholerae releases the CT complex into the small intestine, the B subunits bind to ganglioside receptors on the surface of intestinal epithelial cells. [1]
Once bound, the CT is endocytosed into the host cell and promptly travels to the Golgi and endoplasmic reticulum. Nicking of a protease-sensitive loop in CTA by host or bacterial protease generates the CT-A1 polypeptide. [4] The catalytic CT-A1 polypeptide leaves the ER and enters the cytosol by retro-translocation, where it activates the Gsα subunit of the guanine nucleotide-binding regulatory (Gαs) protein. [1]
Activation of the Gαs-protein increases adenylate cyclase (AC) activity. AC cleaves ATP to cyclic adenosine monophosphate (cAMP), resulting in uncontrolled increases in intracellular cAMP. Elevated cAMP levels disrupt ion transport mechanisms in the intestinal epithelium. [1]
cAMP activates protein kinase-A (PKA), which inhibits NaCl absorption through the Na+/H+ exchanger and phosphorylates the cystic fibrosis transmembrane conductance regulator (CFTR) chloride channel proteins. [1] This leads to the efflux of chloride ions, HCO3-, Na+, K+, and water into the intestinal lumen. Excessive fluid loss manifests clinically as watery diarrhea, a hallmark symptom of cholera. If left untreated, severe dehydration and electrolyte imbalances can occur, leading to shock, organ failure, and death.
Accessory Toxins
In addition to the cholerae toxin, the accessory cholerae enterotoxin (Ace) and zonula occludens toxin (Zot) contribute to V. cholerae's pathogenesis by inducing changes in the intestinal barrier. Ace appears to cause initial intestinal fluid secretion during infection before the slow-acting CT can take effect. Secretion by Ace utilizes Ca2+ as a second messenger rather than cAMP [5]. However, a comprehensive study on Ace's role in pathophysiology is still lacking.
On the other hand, Zot affects the structure of epithelial tight junctions, which serve as a continuous intercellular barrier between epithelial cells. Zot binds to a cell's α-1-chimaerin receptor and reduces its actin filaments, thus modifying its cytoskeleton and tight junction complex [6]. This modification increases mucosal permeability [7].
Hemagglutinin Protease
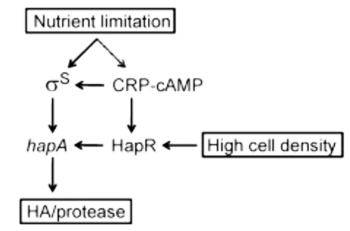
V. cholerae expresses Hemagglutinin/protease (HA/protease) encoded for by the hapA gene. Expression of hapA is complex and concerns various environmental signals and regulators. Transcription of hapA requires the quorum sensing regulator HapR, the cyclic AMP receptor protein (CRP), and the RpoS alternative sigma factor. hapA transcription activates under limited nutrient conditions, at high cell population density, and in stationary phase [8].
Nutrient limitation results in elevated cAMP levels and activates RpoS and CRP [8]. Activation of CRP further enhances rpoS transcription and also activates HapR expression. HapR directly and indirectly activates the transcription of hapA by increasing RpoS expression [9]. Therefore, nutritional and population density signals are often coordinated [10][11].
V. cholerae secretes HA/protease through the type II secretion (T2SS) pathway. HA/protease is involved in several pathogenic activities, including the modification of other toxins and the degradation of the protective mucus barrier in the gut [8]. HA/protease induces hemorrhagic responses, leading to the degeneration of laminin and collagen in vascular endothelial cells. It cleaves occludin. This causes the F-actin cytoskeleton to reorganize and disrupts the paracellular barrier function, which is responsible for maintaining the integrity of the intestinal lining [12].
Additionally, HA/protease aids in the formation of biofilms, assists in the delivery of cholera toxin (CT), and could contribute to the activation of the CT-A subunit. As a result, HA/protease has multiple targets during infection, which can increase the severity of the illness [8].
Hemolysin Toxin
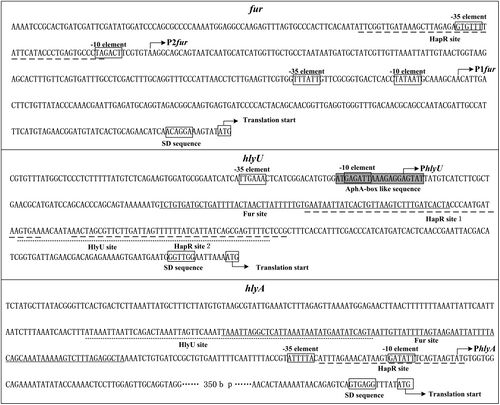
DNA Regulation
V. cholerae O1 has a hlyA gene on Chr 2 that codes for the hemolysin toxin (HlyA). HlyA production is controlled by quorum sensing, which is mediated by HapA at the post-translation level [13].
HlyA expression is regulated by quorum sensing and regulatory proteins HapR, HlyU, and Fur [14]. During early-to-mid-log phase, Fur binds to the promoters of hlyU and hlyA to suppress their transcription. Simultaneously, HlyU binds to the promoters of hlyA and hlyU to activate and inhibit their transcription, respectively. In late log phase, HapR binds to the Fur, HlyU, and HlyA promoters to suppress their transcription [14].
A study conducted in 2018 suggested that the intricate regulation system of hlyA is advantageous for different stages of V. cholerae pathogenesis and infection [14]. During the early to middle stages of infection, V. cholerae releases large amounts of HlyA in the small intestine, leading to more severe watery diarrhea [14]. As the infection progresses, HapR becomes highly expressed. HapR suppresses hlyA, stimulates protease production, prevents the formation of biofilms, and causes the biofilm of V. cholerae cells to disintegrate [14].
HlyA Toxin
V. cholerae O1 El Tor produces HlyA, an extracellular pore-forming toxin. Hemolysin causes blood cell lysis and cytoplasmic vacuolization [14]. HlyA promotes bacterial invasion in the early stages of infection [15]. It possesses a range of biological activities, including hemolytic activity, lethality, cardiotoxicity, cytotoxicity, and enterotoxicity [16]. When injected into rabbit ileal loops, purified HlyA can induce fluid accumulation and alter the mucosa [17]. In vitro studies have shown that HlyA can cause cell vacuolation and apoptosis in cultured mammalian cells [18][19]. Furthermore, HlyA is believed to cause lethality, developmental delays, and intestinal vacuole formation in Caenorhabditis elegans infected with V. cholerae [14] [20] [21].
Toxin-Coregulated Pilus
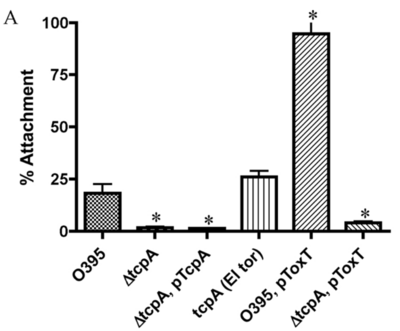
TCP Function
The colonization of the human small intestine by Vibrio cholerae is an important step in the pathogenesis of the disease, which requires the type IV toxin-coregulated pilus (TCP). To date, scientists have identified three functions of TCP: it acts as the CTXΦ receptor, secretes the colonization factor TcpF (a soluble factor essential for colonization in the infant mouse cholera model), and plays a vital role in microcolony formation by facilitating bacterium-bacterium interactions [22].
Mutants that lack TCP are defective in attachment compared to the wild type [22]. Overexpression of ToxT, which is the activator of TCP, significantly increases the attachment of wild-type V. cholerae to Caco-2 cells [22]. Researchers have observed the TCP-mediated attachment of V. cholerae to the small intestines of infected infant mice [23]. Hence, it is clear that type IV toxin-coregulated pilus (TCP) is essential for the successful colonization of the host's small intestines.
TCP Biosynthesis
The production of TCP relies on the tcp operon, a group of 12 genes. The first gene in the operon, tcpA, creates the main pilin subunit of TCP, which is a 20-kDa protein [24]. TcpA is initially expressed as a preprotein and then matures after being cleaved by tcpJ, the prepilin peptidase (an enzyme) [25].
ToxT, a member of the AraC family, activates tcp expression by using signals from a transcriptional cascade known as the toxR regulon [22]. Aside from tcpF, the remaining genes in the operon encode different components of the TCP apparatus, which is responsible for creating the pilus by spanning the inner membrane, periplasm, and outer membrane. TCP production is energy-dependent [22]. The 10th gene in the operon, tcpF, creates a 36-kDa protein that is secreted by the TCP apparatus and is essential for colonizing infant mice [22]. Additionally, disulfide bond formation of the functional pilin subunit requires dsbA, a periplasmic enzyme encoded by the chromosomal dsbA gene [26].
Conclusion
Vibrio cholerae is a gram-negative bacterium associated with cholera epidemics. It is highly motile and survives and transmits via contaminated reservoirs. The bacterium produces several virulence factors, including cholera enterotoxin, hemolysin toxin, and HA/protease, which enable it to colonize hosts and counter their immune responses [1].
The cholera toxin is the primary virulence factor and is responsible for the hallmark symptom of cholera, which is watery diarrhea. Hemagglutinin/protease (HA/protease) is another virulence factor that activates under limited nutrient conditions and at high cell population density. HA/protease can cause several pathogenic activities, including the modification of other toxins, degradation of the protective mucus barrier in the gut, and induction of hemorrhagic responses. It also aids in the formation of biofilms and assists in the delivery of cholera toxin [8].
Hemolysin causes blood cell lysis, cytoplasmic vacuolization, and other harmful effects such as lethality, cardiotoxicity, and enterotoxicity. It is believed to promote bacterial invasion in the early stages of infection and cause fluid accumulation and mucosal changes when injected into rabbit ileal loops. Hemolysin also causes cell vacuolation and apoptosis in cultured mammalian cells and leads to lethality, developmental delays, and intestinal vacuole formation in Caenorhabditis elegans infected with V. cholerae [14]. The type IV toxin-coregulated pilus (TCP) is important for the pathogenesis of the disease. It facilitates bacterium-bacterium interactions and is essential for intestinal colonization [22].
Cholera has been a public health concern for centuries. Despite sanitation and medical advancements, cholera remains a threat in many areas of the world, particularly those with inadequate sanitation and poor access to clean water. Cholera outbreaks occur regularly in parts of Asia, Africa, Latin America, and the Middle East. Proper sanitation, clean water, Surveillance systems, and prompt treatment with rehydration therapy are essential for managing and preventing cholera outbreaks.
References
- ↑ 1.0 1.1 1.2 1.3 1.4 1.5 1.6 1.7 Ramamurthy, T. N.,et al. “Virulence Regulation and Innate Host Response in the Pathogenicity of Vibrio cholerae." Frontiers in Cellular and Infection Microbiology, Vol. 10, 2020.
- ↑ 2.0 2.1 2.2 2.3 Heidelberg, J. F.,et al. “DNA sequence of both chromosomes of the cholera pathogen Vibrio cholerae.” Nature, Vol. 406, Issue 6795, 2000, pp. 477–483.
- ↑ Casadevall, Arturo and Pirofski, Liise-anne. "Virulence factors and their mechanisms of action: the view from a damage–response framework." Journal of Water and Health, Vol. 7, Issue S1, 2009, pp. S2–S18.
- ↑ Jobling, G. Michael and Holmes, K. Randall. "Identification of motifs in cholera toxin A1 polypeptide that are required for its interaction with human ADP-ribosylation factor 6 in a bacterial two-hybrid system." Proceedings of the National Academy of Sciences of the United States of America, Vol. 97, Issue 26, 2000, pp. 14662–14667.
- ↑ Trucksis, M., et.al. “Vibrio cholerae ACE stimulates Ca(2+)-dependent Cl(-)/HCO(3)(-) secretion in T84 cells in vitro." American Journal of Physiology-Cell Physiology, Vol. 279, No. 3, 2020, pp. C567-C577.
- ↑ Uzzau, S., et al. “Purification and preliminary characterization of the zonula occludens toxin receptor from human (CaCo2) and murine (IEC6) intestinal cell lines." FEMS Microbiology Letters, Vol. 194, Issue 1, 2001, pp. 1–5.
- ↑ Goldblum, E. Simeon., et.al. “The active Zot domain (aa 288–293) increases ZO-1 and myosin 1C serine/threonine phosphorylation, alters interaction between ZO-1 and its binding partners, and induces tight junction disassembly through proteinase activated receptor 2 activation." Federation of American Societies for Experimental Biology, Vol. 25, Issue 1, 2011, pp. 144–158.
- ↑ 8.0 8.1 8.2 8.3 8.4 Benitez, A. Jorge and Silva J. Anisia. “Vibrio cholerae hemagglutinin(HA)/protease: An extracellular metalloprotease with multiple pathogenic activities." Toxicon, Vol. 115, 2016, pp. 55–62.
- ↑ Wang, Liang et.al. “Cyclic AMP (cAMP) and cAMP Receptor Protein Influence both Synthesis and Uptake of Extracellular Autoinducer 2 in Escherichia coli." American Society for Microbiology Applied and Environmental Microbiology, Vol. 187, Issue 6, 2005, pp. 2066–2076.
- ↑ Benitez, A. Jorge, et.al. “Environmental Signals Controlling Production of Hemagglutinin/Protease in Vibrio cholerae." American Society for Microbiology Infection and Immunity, Vol. 69, Issue 10, 2001, pp. 6549–6553.
- ↑ Liang, Weili. “Cyclic AMP post-transcriptionally regulates the biosynthesis of a major bacterial autoinducer to modulate the cell density required to activate quorum sensing." FEBS Letters, Vol. 582, Issue 27, 2008, pp. 3744–3750.
- ↑ Förster, Carola et.al. “Differential effects of hydrocortisone and TNFα on tight junction proteins in an in vitro model of the human blood–brain barrier." The Journal of Physiology, Vol. 586, Issue 7, 2005, pp. 1937–1949.
- ↑ Tsou, A. M., & Zhu, J. (2010). Quorum sensing negatively regulates hemolysin transcriptionally and posttranslationally in Vibrio cholerae. Infection and immunity, 78(1), 461–467.
- ↑ 14.0 14.1 14.2 14.3 14.4 14.5 14.6 14.7 Gao, H., Xu, J., Lu, X., Li, J., Lou, J., Zhao, H., Diao, B., Shi, Q., Zhang, Y., & Kan, B. (2018). Expression of Hemolysin Is Regulated Under the Collective Actions of HapR, Fur, and HlyU in Vibrio cholerae El Tor Serogroup O1. Frontiers in microbiology, 9, 1310.
- ↑ Diep, T. T., Nguyen, N. T. N., Nguyen, T. N. C., An, H. K., Nguyen, T. Q., Nguyen, V. H., Nguyen, T. V., Nguyen, T. N. A., Izumiya, H., Ohnishi, M., Yamashiro, T., & Nguyen, L. T. P. (2015). Isolation of New Delhi metallo‐β‐lactamase 1‐producing Vibrio cholerae non‐O1, non‐O139 strain carrying ctxA, st and hly genes in southern Vietnam. In Microbiology and Immunology (Vol. 59, Issue 5, pp. 262–267)
- ↑ Benitez, J. A., & Silva, A. J. (2016). Vibrio cholerae hemagglutinin(HA)/protease: An extracellular metalloprotease with multiple pathogenic activities. In Toxicon (Vol. 115, pp. 55–62). Elsevier BV.
- ↑ Ichinose, Y., Yamamoto, K., Nakasone, N., Tanabe, M. J., Takeda, T., Miwatani, T., & Iwanaga, M. (1987). Enterotoxicity of El Tor-like hemolysin of non-O1 Vibrio cholerae. Infection and immunity, 55(5), 1090–1093.
- ↑ Mitra, R., Figueroa, P., Mukhopadhyay, A. K., Shimada, T., Takeda, Y., Berg, D. E., & Nair, G. B. (2000). Cell vacuolation, a manifestation of the El tor hemolysin of Vibrio cholerae. Infection and immunity, 68(4), 1928–1933.
- ↑ Coelho, A., Andrade, J. R., Vicente, A. C., & Dirita, V. J. (2000). Cytotoxic cell vacuolating activity from Vibrio cholerae hemolysin. Infection and immunity, 68(3), 1700–1705.
- ↑ Sahu, S. N., Lewis, J., Patel, I., Bozdag, S., Lee, J. H., LeClerc, J. E., & Cinar, H. N. (2012). Genomic analysis of immune response against Vibrio cholerae hemolysin in Caenorhabditis elegans. PloS one, 7(5), e38200.
- ↑ Cinar, H. N., Kothary, M., Datta, A. R., Tall, B. D., Sprando, R., Bilecen, K., Yildiz, F., & McCardell, B. (2010). Vibrio cholerae hemolysin is required for lethality, developmental delay, and intestinal vacuolation in Caenorhabditis elegans. PloS one, 5(7), e11558.
- ↑ 22.0 22.1 22.2 22.3 22.4 22.5 22.6 Krebs, S. J., & Taylor, R. K. (2011). Protection and attachment of Vibrio cholerae mediated by the toxin-coregulated pilus in the infant mouse model. Journal of bacteriology, 193(19), 5260–5270.
- ↑ Taylor, R. K., Miller, V. L., Furlong, D. B., & Mekalanos, J. J. (1987). Use of phoA gene fusions to identify a pilus colonization factor coordinately regulated with cholera toxin. Proceedings of the National Academy of Sciences of the United States of America, 84(9), 2833–2837.
- ↑ Megli, C. J., & Taylor, R. K. (2013). Secretion of TcpF by the Vibrio cholerae toxin-coregulated pilus biogenesis apparatus requires an N-terminal determinant. Journal of bacteriology, 195(12), 2718–2727.
- ↑ LaPointe, C. F., & Taylor, R. K. (2000). The type 4 prepilin peptidases comprise a novel family of aspartic acid proteases. The Journal of biological chemistry, 275(2), 1502–1510.
- ↑ Peek, J. A., & Taylor, R. K. (1992). Characterization of a periplasmic thiol:disulfide interchange protein required for the functional maturation of secreted virulence factors of Vibrio cholerae. Proceedings of the National Academy of Sciences of the United States of America, 89(13), 6210–6214.
Authored for BIOL 238 Microbiology, taught by Joan Slonczewski,at Kenyon College,2024