Central Metabolism (Flooded soils): Difference between revisions
Line 23: | Line 23: | ||
====Redox Potential (E<sub>h</sub>)==== | ====Redox Potential (E<sub>h</sub>)==== | ||
Redox potential is the tendency for a reaction, specifically the movement and transfer of electrons, to occur spontaneously and is reported as E<sub>h</sub> in mV. These measurements have been experimentally determined through aqueous solutions containing electrodes, one being the cathode (electron donator) and one being the anode (electron receiver). <ref>Redox Chemistry Primer. (n.d.). Retrieved March 12, 2016, from http://www.kgs.ku.edu/Hydro/GWtutor/Plume_Busters/remediate_refs/redox_chemistry.htm .</ref> | Redox potential is the tendency for a reaction, specifically the movement and transfer of electrons, to occur spontaneously and is reported as E<sub>h</sub> in mV. These measurements have been experimentally determined through aqueous solutions containing electrodes, one being the cathode (electron donator) and one being the anode (electron receiver). <ref>Redox Chemistry Primer. (n.d.). Retrieved March 12, 2016, from http://www.kgs.ku.edu/Hydro/GWtutor/Plume_Busters/remediate_refs/redox_chemistry.htm .</ref> | ||
This voltage shows how likely the electrons will be moved in a solution. Redox potential is assigned individually to half-reactions (a single instance of oxidation or reduction), e.g. the E<sub>h</sub> of the reduction of O<sub>2</sub> to H<sub>2</sub>O will be different from that of the opposite oxidation of H<sub>2</sub>O to O<sub>2</sub>. Redox potential is also reported as standard reduction potential E<sub>o</sub>. Reactions with a higher redox potential yield more net energy for the organism performing them, and this results in higher growth rates (in terms of population). | This voltage shows how likely the electrons will be moved in a solution. Redox potential is assigned individually to half-reactions (a single instance of oxidation or reduction), e.g. the E<sub>h</sub> of the reduction of O<sub>2</sub> to H<sub>2</sub>O will be different from that of the opposite oxidation of H<sub>2</sub>O to O<sub>2</sub>. Redox potential is also reported as standard reduction potential E<sub>o</sub>. Reactions with a higher redox potential yield more net energy for the organism performing them, and this results in higher growth rates (in terms of population). | ||
===The Electron Tower=== | ===The Electron Tower=== | ||
Line 33: | Line 33: | ||
O<sub>2</sub>, the lowest oxidizing agent on the tower, yields the most energy when reduced in a redox reaction with a specific electron donor and will be the first electron acceptor depleted when commonly available. In flooded soils, the amount of oxygen in the system will be very small. When the soil’s microbial population exhausts its remaining O<sub>2</sub>, it will begin using available electron acceptors which provide the next highest amount of energy. Competition generally limits the use of weaker electron acceptors; for example, iron reducers (using Fe<sup>3+</sup>) may exist in the soil but will be dominated by the presence of denitrifiers, who will growth faster with access to their stronger electron acceptor (NO<sub>3</sub><sup>-</sup>). Overall, this process of succession will continue as each electron acceptor supply is used. | O<sub>2</sub>, the lowest oxidizing agent on the tower, yields the most energy when reduced in a redox reaction with a specific electron donor and will be the first electron acceptor depleted when commonly available. In flooded soils, the amount of oxygen in the system will be very small. When the soil’s microbial population exhausts its remaining O<sub>2</sub>, it will begin using available electron acceptors which provide the next highest amount of energy. Competition generally limits the use of weaker electron acceptors; for example, iron reducers (using Fe<sup>3+</sup>) may exist in the soil but will be dominated by the presence of denitrifiers, who will growth faster with access to their stronger electron acceptor (NO<sub>3</sub><sup>-</sup>). Overall, this process of succession will continue as each electron acceptor supply is used. | ||
===Succession of Electron Acceptors=== | ===Succession of Electron Acceptors=== | ||
Line 48: | Line 49: | ||
Methane production: CO<sub>2</sub> + 8 H<sup>+</sup> + 8e<sup>-</sup> -> CH<sub>4</sub> +2 H<sub>2</sub>O (by methanogens) | Methane production: CO<sub>2</sub> + 8 H<sup>+</sup> + 8e<sup>-</sup> -> CH<sub>4</sub> +2 H<sub>2</sub>O (by methanogens) | ||
====[[Nitrogen Cycle|Nitrate Reduction]]==== | ====[[Nitrogen Cycle|Nitrate Reduction]]==== |
Revision as of 04:40, 14 March 2016
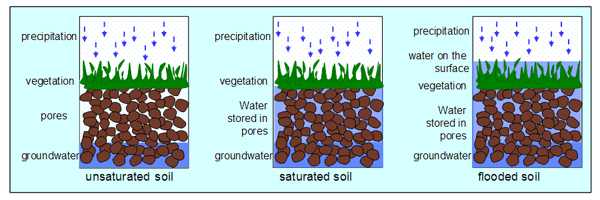
Flooded soils are a condition in which soil is oversaturated with water, often due to natural occurrence or with intended purpose for agricultural reasons. Perpetually flooded soils can be found in wetlands, swamps and marshes; temporary flooded soils can be an effect of season weather or agricultural practices. As water levels fluctuate, and soils alternate between flooded and unflooded states, the chemical makeup of the soil environment will continuously change. A soil’s water content directly influences both inorganic and microbial reactions that affect the soil’s redox potential (Eh), acidity, alkalinity, and salinity.[1]
One of the most important effects of flooding is that the presence of oxygen is limited in such an environment, and any remaining oxygen is quickly used up via aerobic respiration. As a result, other compounds are used as electron acceptors in energy acquisition reactions; the microorganisms that specialize in conducting these other reactions are able to flourish and affect nutrient cycling in the ecosystem.
Microbial transformations of elements in anaerobic soils play a worldwide role in biogeochemical cycling of nutrients and in greenhouse gas emissions. Changes in the oxidation state of terminal electron acceptors may result in nutrient loss from the system via volatilization or leaching. Anaerobic microbial processes including denitrification, methanogenesis, and methanotrophy are responsible for releasing greenhouse gases (N2O, CH4, CO2) into the atmosphere. [2]
Key Microbial Processes
Microbial Respiration: Oxidation/Reduction (Redox) Reactions
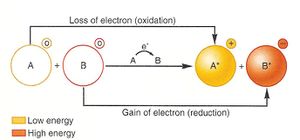
In order to obtain energy, many microbes make use of the process of respiration through a redox reaction. Respiration is a catabolic reaction that produces ATP in which either organic or inorganic compounds act as primary electron donors and exogenous compounds act as the terminal electron acceptors. In a redox reaction, one molecule (the reducing agent) loses electrons and another molecule (the oxidizing agent) accepts electrons. Electron donors such as glucose, methanol, and NADH are energy sources that can be thought of as “giving up” their electrons, while another molecule is need to receive said electrons. For example, in aerobic respiration, energy rich compounds like glucose (the reducing agent) are oxidized to carbon dioxide, with oxygen (the oxidizing agent) acting as a terminal electron acceptor and being reduced to water. In addition to oxygen, microorganisms use a large variety of electron acceptors.
Depending on the type of electron acceptors used by microorganisms, microbes can be placed into a variety of classifications. Strict aerobes can only use oxygen as a terminal electron acceptor. Obligate anaerobes cannot use oxygen and are actually inhibited or poisoned by oxygen. Facultative anaerobes are flexible in electron acceptor usage; as a result of this they can make use of other redox reactions to maintain a supply of energy as oxygen levels decrease.
Oxygen gas (O2) is one of the most favorable electron acceptors, but it is typically not available in flooded soils. Instead, facultative and strict anaerobic microbes utilize other oxidizing agents/electron acceptors to carry out respiration. The amount of energy that can be obtained through respiration varies between compounds and microbes and will make use of these compounds in order of decreasing redox potential, thus leading to a succession of acceptors.
Redox Potential (Eh)
Redox potential is the tendency for a reaction, specifically the movement and transfer of electrons, to occur spontaneously and is reported as Eh in mV. These measurements have been experimentally determined through aqueous solutions containing electrodes, one being the cathode (electron donator) and one being the anode (electron receiver). [4]
This voltage shows how likely the electrons will be moved in a solution. Redox potential is assigned individually to half-reactions (a single instance of oxidation or reduction), e.g. the Eh of the reduction of O2 to H2O will be different from that of the opposite oxidation of H2O to O2. Redox potential is also reported as standard reduction potential Eo. Reactions with a higher redox potential yield more net energy for the organism performing them, and this results in higher growth rates (in terms of population).
The Electron Tower
Microbes will successively use the highest energy yielding electron acceptors available in the order indicated on the electron tower, which is a ranking of common redox reactions by the amount of energy that can be obtained from them. Compounds are listed in redox pairs (oxidized form and reduced form) The greater the difference in electrical potential between the reactants and products of a reaction the the greater release of energy that is crucial for microbial growth. [5]
O2, the lowest oxidizing agent on the tower, yields the most energy when reduced in a redox reaction with a specific electron donor and will be the first electron acceptor depleted when commonly available. In flooded soils, the amount of oxygen in the system will be very small. When the soil’s microbial population exhausts its remaining O2, it will begin using available electron acceptors which provide the next highest amount of energy. Competition generally limits the use of weaker electron acceptors; for example, iron reducers (using Fe3+) may exist in the soil but will be dominated by the presence of denitrifiers, who will growth faster with access to their stronger electron acceptor (NO3-). Overall, this process of succession will continue as each electron acceptor supply is used.
Succession of Electron Acceptors
In summary, the main succession of electron acceptor usage in flooded soils is as follows:
Aerobic respiration: ½ O2 + 2e- + 2H+ -> H2O (by facultative anaerobes and aerobes)
Denitrification: 2NO3- + 12 H+ +10e- -> N2+6H2O (by denitrifiers)
Manganese reduction: MnO2 + 4H+ + 2e- ->Mn2+ + 2H2O (by manganese reducing bacteria)
Iron reduction: Fe(OH)3 + 3 H+ + 2e- -> Fe2+ + 2H2O (by iron reducing bacteria)
Sulfate reduction: SO42- + 10H+ +8e- -> H2S + 4H2O (by sulfate reducing bacteria)
Methane production: CO2 + 8 H+ + 8e- -> CH4 +2 H2O (by methanogens)
Nitrate Reduction
After O2, nitrate (NO3-) is one of the strongest electron acceptors as is represented in the electron tower. It can be obtained from transformations of other compounds containing nitrogen, such as ammonium (NH4+) and nitrite (NO2-). Denitrification reduces NO3- to nitrogen gas (N2) or various nitrogen oxides and is performed by facultative anaerobic microorganisms. Oxygen depletion is important for the nitrogen cycle as a whole, since if it were constantly present NO3- would be used at a much slower rate and contaminate soils through accumulation. [6]
Manganese Reduction
The next most energy-releasing electron acceptor after NO3- is manganese (IV) oxide (MnO2) which is is reduced to Mn2+ ions. In this form, manganese is very insoluble in water and forms masses in soils. Mn2+ is generally oxidized to this form in soils with a pH between 5 and 8, (as the rate increases with basicity). Many microorganisms that conduct this process are also capable of iron reduction, described below. [6]
Iron Reduction
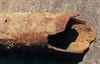
The utilization of ferric iron ions (Fe3+), at approximately 120 mV, occurs when ions are released from metal deposits or minerals in the soil. Ferrous iron (Fe2+) product causes soil gleying, a process described below, when it accumulates. The source of the iron is also of relevance; whereas iron reduction in phosphate minerals can release phosphate for other organisms, it can also lead to corrosion of steel where iron reducers are present. Some Pseudomonas species can release pseudobactin, an iron-binding compound that limits its availability to other bacteria. [6]
Sulfate Reduction
Sulfate reduction begins occurring at 0 mV, and the dissimilatory reduction results in hydrogen sulfide (H2S) being released. However, H2S is prone to reaction with Fe2+ to form iron sulfide (FeS). As a result, it often reacts before it reaches the surface of the soil, unable to disperse into the air. [6] Sulfate reduction has several documented consequences, ranging from corrosion of underground iron pipes due to FeS formation and blackening of soil caused by liberation of organic matter. Hydrogen sulfide is known as “swamp gas”, due to its emergence from one form of soil flooding, and has an odor comparable to rotting eggs. It pollutes many bodies of water and the air above them; due to its flammability and toxicity, this is very dangerous. [7]
Methanogenesis (by Carbon Dioxide Reduction)
The process of using carbon dioxide (CO2) as a terminal electron acceptor results in the formation of methane (CH4) and is known as methanogenesis. In soil, it occurs almost exclusively in a flooded condition due to its reduction potential being so low (below -100 mV). The use of CO2 in this fashion yields much less energy than the reactions of previous electron acceptors, so this process has lower growth rates in turn. Organisms who perform methanogenesis are known as methanogens and are a group of anaerobic Archaea. CH4 can also be produced as a result of acetate (CH3COOH) fermentation, which is also performed by methanogens. [6]
Fermentation in Flooded Soils
Fermentation, a different form of metabolism from respiration, occurs in the absence of a suitable terminal electron acceptor. Cells convert NADH and pyruvate from the glycolysis of sugars into NAD+ and other compounds depending on the species fermenting. The various products of fermentation, including alcohols, lactic acid, and acetate are released into the surrounding soil and then become available for use by other anaerobic organisms. Additionally, fermentation generally reduces soil pH, which will encourage dissolution of minerals and their subsequent access by bacteria. [8]
Microorganisms Involved
Effects of Flooding on Soil Environment
Mobility of Minerals and Gasses
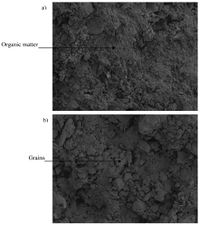
Water also acts as a solvent for ions and soluble compounds, thus increasing the mobility and availability of metal ions, nutrients, and minerals. As a result of these physical effects in flooded soils, microbial respiration will have improved access to water-soluble compounds such as nitrate, perchlorate, manganese/ferric iron, sulfate, and carbon dioxide to act as electron acceptors.
The diffusion of oxygen is over 10000 times slower in water than it is in air at standard temperature and pressure, resulting in the replenishing rate being much slower rate than microbial respiration. Carbon dioxide is also decreased but to a lesser degree due to it being far more soluble than oxygen. [9]
Flooded Soil Aggregate Structure
Though some degree of moisture is important for aggregate formation and microbial activity, flooded soils exhibit decreased aggregate stability. Oxygen depletion and subsequent use of various elements for redox contributes to this decreased aggregation. Organic carbon is also made more soluble (like metals and minerals) under reducing conditions. The decreased stability of the soil is unlikely to recover due to drainage or volatilization of chemicals, removing them from the local environment. Since areas such as marshlands and rice fields are perpetually flooded, their soil’s aggregate stability rarely improves. [10]
Soil Gradients
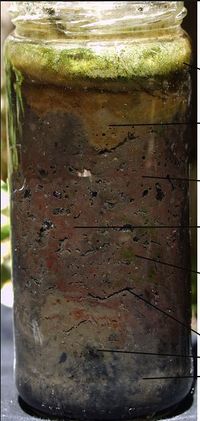
The progression of electron acceptor utilization occurs at different rates in different layers of soil. A process like methanogenesis will occur earlier several feet underground than at the surface due to decreased access to other compounds. This will result in gradients at various depths of soil. These gradients will differ by pH, color, chemical prevalence, and microbial population.
Since the soil is a more closed system (with less mobility of chemicals) than the surface, air, or bodies of water, gradients can be observed with a similar closed system: a Winogradsky column. This column, consisting of a sealed column of soil with provided organic material (such as an egg), allows for simulation of an anaerobic soil environment. [11]
Variation of pH
pH has a major influence over the dissolution and sorption of several important toxins and nutrients in the soil. Low pH values increase the solubility of free aluminum (Al3+) and iron (Fe3+ and Fe2+) ions which can be toxic in high concentrations, while also reducing the availability of phosphorus.
When soil is initially saturated with water, the pH drops due to the accumulation of carbonic acid formed from trapped carbon dioxide produced from respiration. Fermentation also contributes to pH decreasing through the production of organic acids. This is quickly followed by an increase of pH as hydrogen cations are consumed in microbially-driven redox reactions. The soil then will gradually approach and stabilize near a neutral pH, with pH increasing in acidic soil and decreasing in basic soil due to products such as carbonate forming a buffer. [12]
Soil Gleying
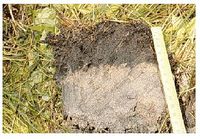
Gleying is a phenomenon in which waterlogged soils are discolored by accumulation of Iron Oxide (FeO) due to reduction of ferric iron into ferrous iron. Although ferric iron exists as an insoluble form in flooded soils, more ferrous iron can accumulate by the reduction of ferric iron over time. This results in a greenish, blue, grey soil color. In general Fe3+ -reducing fermentative bacteria can be readily isolated from gleyed soils. The black color of soils/solution is frequently observed in flooded soil. This may result from the formation of iron sulfides (FeS) and pyrite (FeS2). [13]
Plant Nutrient Availability
Flooded soils can have a direct impact on plants by preventing efficient gas exchange between the plant roots and the soil. These conditions also change the types of microbes that are active and what they produce. Flooded soils cause anaerobic conditions which forces many microbes to use less favorable electron acceptors. These less favorable acceptors could have also served as nutrition for the plants; this creates competition between the plants and the microbes in certain flooded soils. By using different electron acceptors the microbes will release different products causing a chemical change in the soil that can be toxic. [14]
Flooded to Unflooded Conditions
When waterlogged soils drain, oxygen diffuses into soil pores. The soil’s Eh increases and aerobic activity kicks in ceasing the production of anaerobic products. At higher Eh zones ( > 500 mV), undecomposed soil organic matter is used as an electron donor by aerobes and converted to water and CO2. Many of the anaerobic redox reactions are also reversed as with oxygen available as an electron acceptor many of the reduced products of anaerobic respiration can now be used as electron donors by lithotrophs. Manganese is oxidized back to MnO2 which gives some aerated soils a black color, and ferrous iron is oxidized by an iron-oxidizing bacteria, resulting in the formation of ferric oxides or ferric hydroxide minerals that give the soil a red, yellow, or brownish texture. [8]
Processes
Eh
During the succession of anaerobic oxidation processes, the redox potential (Eh) of flooded soils will decrease as a result of the reduced products formed. Approximate values for redox potentials associated with specific oxidation-reduction process are as follows:
Observation | Eh (mV) |
Disappearance of oxygen | +330 |
Disappearance of nitrate | +220 |
Appearance of manganese ions | +200 |
Appearance of ferrous iron ions | +120 |
Disappearance of sulfate | -150 |
Appearance of methane | -250 |
Micro Involved
Microbial processes
Microbial Activity
In anaerobic respiration oxygen is replaced by other compounds as TEA (terminal electron acceptors). Some important terminal electron acceptors include iron, nitrate, sulfate, and manganese. These processes are primarily driven by microbial activity. Energy yields of alternative electron acceptors are lower than that of aerobic respiration, in which oxygen is utilized as a TEA (see #Electron Tower theory. As available oxygen declines, organisms that thrive under anoxic conditions proliferate using alternative electron acceptors. The order in which available electron acceptors are consumed can generally be predicted by the electron tower and associated energy yields of electron pairs. Changes in redox conditions of flooded soils over time reflects the successive availability of TEA's from the electron tower, and will govern which microbes will thrive, as those able to use these available alternative electron acceptors. Flooding also alters microbial flora in soil by decreasing the O2 concentration. Fermentation is a major biochemical processes responsible for organic matter decomposition in flooded soils. Eh levels can affect which compounds are fermented. These levels will tend to gradually drop in flooded soils.
Fermentation under Anoxic Conditions
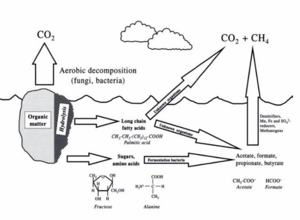
There are many types of fermentative bacteria in soils, such as the genus Bacillus, Clostridium, and Lactobacillus. 4 ATP molecules per molecule of glucose are produced by fermentation, while 38 ATP molecules are produced by aerobic respiration. Although the energy yield via fermentation is less than respiration, fermentation plays an important role in anaerobic respiration for obligate and facultative anaerobic bacteria, including denitrifier, Fe3+, Mn4+, SO42-, reducers, and methanogens. Sugar (glucose or fructose) is broken down into simple compounds (e.g. formate, acetate, and ethanol) during fermentation. Also, numerous fermentation products, such as carbon dioxide, fatty acid, lactic, alcohols, are released into soils. These compounds serve as substrates for other anaerobic bacteria. Thus, low molecular weight organic compounds produced from fermentation influence the reduction of Fe(III), Mn(IV), SO42-, and CO2(Richardson And Vepraskas 2000).
Organisms involved in Flooded Soils
Nitrate Reducing Bacteria
When available oxygen is depleted and nitrate is available, denitrification, the reduction of NO3- to NO,N2O,or N2, primarily occurs. Denitrification is carried out by obligate respiratory bacteria belonging to the genera Agrobacterium, Alcaligenes, Bacillus, Paracoccus denitrificans, Pseudomonas and Thiobacillus (Knowles, 1982). Nitrate ammonification found in facultative anaerobic bacteria belonging to the genera Bacillus, Citrobacter and Aeromonas, or members of the Enterobacteriaceae (Cole and Brown, 1980; Smith and Zimmerman, 1981; MacFarlane and Herbert, 1982). Strictly anaerobic bacteria belonging to the genus Clostridium are also able to reduce nitrate to ammonia (Hasan and Hall, 1975). Pure culture studies show evidence that nitrate reduction may also occur in the presence of oxygen (Kuenen and Robertson, 1987).
Iron/Manganese Reducing Bacteria
Most microorganisms can reduce Mn4+ and Fe 3+. Ferrous iron is used as electron acceptor by iron-reducing bacteria such as Geobacter(Geobacter metallireducens and Geobacter sulfurreducens),Shewanella putrefaciens,Desulfovibrio, Pseudomonas, and Thiobacillus(Lovley 1993). Bacillus, Geobacter, and Pseudomonas are representative manganese-reducing bacteria. Different forms of ferric iron oxides exist in drained aerobic soils as well as in waterlogged soils. Not all forms of ferric oxides are equally suitable for reduction by ferric oxide reducer bacteria (Gotoh and Patrick, 1974; Schwertmann and Taylor, 1977). In general, amorphous forms are more efficient for ferric reducer bacteria than crystalline forms (Lovely and Phillips, 1986). The reduction of ferric oxide may release phosphate and trace elements that are adsorbed to amorphous ferric oxide and thus enhance availability of these compounds in the soil (Lovely and Phillips, 1986).
Sulfate Reducing Bacteria
Bacteria can use organic compounds as an electron donor and sulfate as an electron acceptor. This reaction for acetate as electron donor is as follows:
CH3COO- + SO42- + 3 H+ ---> 2CO2 + H2S + 2 H2O
This reaction is carried out by sulfate-reducing bacteria such as Desulfobacter, Desulfobulbus, Desulfococcus, Desulfovibrio, Desulfosarcina,Desulfotomaculum,and Desulfonema(Langston and Bebiano 1998, Sylvia 2004). Some of the sulfate reducing bacteria oxidizes the organic compounds completely to CO2 and some other stop after producing acetate as an intermediate of oxidation. Hydrogen sulfide gas produced via anaerobic respiration causes the rotten egg odor.
Recently, Boetius et al and Orphan et al revealed that a consortium of Desulfosarcina and methanotrophic archaea in the sediments contribute to control methane gas.
CH4 +SO4 2------->HCO3+HS -+H2O
Methanogens
Methanogen products less energy than other reducing reaction because the reduction of carbon dioxide occur under the most anaerobic and reduced conditions(see #Electron tower section). Thus, the activity of methanogen is repressed until other alternative terminal electron acceptor such as Fe(III), NO3-,and SO42-, have been depleted.
Methanogen (e.g Methanobacterium formicum, Methanobacterium bryantii, Methanobacterium thermo-autrotrophicum, and etc ) can use CO2 and produce methane (Langston and Bebiano 1998)
Environmental Issues
Flooded soils are dynamic ecosystems that play an important role in biogeochemical cycling and in the production of greenhouse gases. Methane (CH4+) and nitrous oxide (N2O) are produced as byproducts of anaerobic metabolism in the low-redox zones characteristic of flooded soils, where oxygen is lacking. Carbon dioxide (CO2), which receives widespread attention as a greenhouse gas and potential source of global warming, may also be produced at the interface of anaerobic-aerobic zones through the consumption of methane gas. However, it should be noted that from a global standpoint methane and nitrous oxide on a per molecule basis have the potential to contribute 25x and 300x more to global warming over the next century than carbon dioxide, respectively (Schlesinger, 1997). Thus the conversion of methane gas to carbon dioxide essentially reduces the greenhouse gas effect by 25x per molecule per 100 years. According to Matthews and Fung (1987), an estimated 3.6% of terrestrial land is classified as wetlands, and although this number continues to decline (Schlesinger, 1997) the effect of flooded soils to the global climate is clear.
Methane Production; Methanogenesis
Methane production occurs exclusively in anaerobic conditions by a group of Archaea known as methanogens. These microbes are obligatory, and require extremely low redox conditions in the range of -100mV (see #electron tower theory, section 2.1.1) (Sylvia, 2005). If oxygen is introduced into the system, methanogenesis ceases; thus, the process of methanogenesis depends on saturated soil conditions.
Methanogenesis can occur via one of two pathways: either by 1) CO2 reduction or by 2) acetate fermentation.
1) CO2 + H2 --> CH4+ (CO2 reduction)
and
2) CH3COOH --> CH4+ + CO2 (acetate fermentation)
Both acetate and hydrogen are byproducts of anaerobic fermentation.
Because the process of methanogenesis is “fed” byproducts produced from a complex series of degradation processes which are themselves “fed” complex organic matter, rates of methane production are highly sensitive to changes in temperature. Methanogenesis has a Q10 value in the range of 30-40, which is substantially higher than most biochemical process (Sylvia, 2005).
Despite the clear effect of increasing temperatures on the rate of methanogenesis, the actual impact of global warming on methane production rates in wetlands and permafrost regions is highly unpredictable. Because methanogenesis requires anoxic conditions, any drying of flooded soil environments would both decrease methane production and increase methane oxidation, reducing overall methane emissions. Alternatively, warmer climates could increase growing seasons, which would increase methane emissions (Sylvia, 2005).
CO2 Production via Methane Consumption: Methanotrophy
Some of the methane produced via methanogenesis in flooded soils may be consumed and oxidized to CO2 at the interface of the anaerobic-aerobic zones. This process occurs primarily by a group of bacteria known as methanotrophs. These microbes can be found in surface layers of wetland soils and unsaturated upland soils, and may be exposed to very high concentrations of methane gas, sometimes amounting to 10% or more of the dissolved gases. Methane is thought to be the only source of C and energy for these bacteria.
Methanotrophy occurs in the following reaction:
CH4+ + 2O2 --> CO2 + 2H2O
Methane is similar in size and shape to ammonium; and there is some evidence that nitrifiers (ammonium oxidizers) can also oxidize methane (Sylvia, 1998). Because they are molecularly similar, NH4+ competes at the enzyme’s active site, inhibiting methane oxidation. As a result, methanotrophy is generally inhibited by the addition of fertilizer or excess nitrogen in the system, when ammonium levels are high.
Alternatively, if nitrogen is extremely limiting the addition of nitrogen will stimulate methanotrophy and actually increase methane consumption. So although it is generally expected that adding N-fertilizer will decrease CH4+ consumption and lead to increased global warming potential, sometime the opposite effect may occur. (Sylvia, 2005).
Nitrous Oxide; Denitrification
Denitrification is an anaerobic process in which nitrate serves as the terminal electron acceptor, and generally some source of organic carbon is the electron donor (also H2 may serve as a donor).
In this process, nitrate is oxidized to nitric oxide, then nitrous oxide, and then fully oxidized to dinitrogen:
NO2- --> NO --> N2O --> N2
However, under certain conditions the full oxidation of NO3- to N2 does not occur and nitrous oxide (N2O) is produced.
Microbes responsible include both organotrophs and lithotrophs, and this process occurs primarily by facultative anaerobes.
Although a low redox potential is important for denitrification to occur (oxygen must not be present or it will “out-compete” nitrate as a terminal electron acceptor), redox requirements are not so low that this process cannot occur within anaerobic microsites of soil aggregates.
Factors affecting nitrous oxide production include oxygen, pH, and the ratio of nitrate to available C. Although denitrification rates decrease with increasing oxygen, the proportion of N evolved as nitrous oxide actually increases with increasing oxygen. Low pH generally inhibits the reduction of N2O to N2; thus at low pH, N2O will likely dominate. However, highly acidic soils have low N availability and low nitrification and denitrification rates. Thus, the highest rate of nitrous oxide production from denitrification occurs in moist soils that cycle N rapidly (Sylvia, 2005).
Current Research
Current research topics on the issue of flooded soils are heavily focused on greenhouse gas emissions produced as a result of the low redox conditions characteristic of these ecosystems. Other research topics may address impacts to plant growth, and chemical, physical, and biological aspects of flooded soils. Following is a list of recent journal review articles focused on a range of current research topics related to flooded soil environments for the interested reader:
1. Shangping Xu, Peter R. Jaffe and Denise L. Mauzerall, A process-based model for methane emission from flooded rice paddy systems, Ecological ModellingVolume 205, Issues 3-4, , 24 July 2007, Pages 475-491. (http://www.sciencedirect.com/science/article/B6VBS-4NHV759-1/2/3126b5403a44c51c5d8d4160382d848e)
2. HANK GREENWAY , WILLIAM ARMSTRONG , and TIMOTHY D. COLMER Conditions Leading to High CO2 (>5 kPa) in Waterlogged–Flooded Soils and Possible Effects on Root Growth and Metabolism AOBPreview published on July 1, 2006, DOI 10.1093/aob/mcl076. Ann Bot 98: 9-32.
3. Kazunori Minamikawa and Naoki Sakai, The effect of water management based on soil redox potential on methane emission from two kinds of paddy soils in Japan, Agriculture, Ecosystems & EnvironmentVolume 107, Issue 4, , 30 May 2005, Pages 397-407. (http://www.sciencedirect.com/science/article/B6T3Y-4FY9M5H-1/2/98a4074277436af97833c16a823b326c) Keywords: Methane; Water management; Soil redox potential; Oryza sativa L.; Rice yield
4. Makoto Kimura, Jun Murase and Yahai Lu, Carbon cycling in rice field ecosystems in the context of input, decomposition and translocation of organic materials and the fates of their end products (CO2 and CH4), Soil Biology and BiochemistryVolume 36, Issue 9, , September 2004, Pages 1399-1416. (http://www.sciencedirect.com/science/article/B6TC7-4C9G7KP-4/2/ceb77d1803f5d02e51bb773f3163cdcb)
5. Sahrawat KL, Fertility and organic matter in submerged rice soils, Current Science. Volume 88, Issues 3-4, 2005, Pages 735-739.
6. Conrad R, Microbial ecology of methanogens and methanotrophs, Advances in Agronomy. Volume 96, 2007, Pages 1-63.
7. Ralf Conrad, Christoph Erkel and Werner Liesack, Rice Cluster I methanogens, an important group of Archaea producing greenhouse gas in soil, Current Opinion in BiotechnologyVolume 17, Issue 3, , Environmental biotechnology/Energy biotechnology, June 2006, Pages 262-267. (http://www.sciencedirect.com/science/article/B6VRV-4JRVFV8-1/2/babb823ea30e51d7445d6861d7d334aa)
References
- ↑ Dassonville, F., & Renault, P. (2002). Interactions between microbial processes and geochemical transformations under anaerobic conditions: A review. Agronomie, 22(1), 51-68. Retrieved from http://www.agronomy-journal.org/articles/agro/abs/2002/01/05/05.html.
- ↑ United States Environmental Protection Agency. (n.d.). Overview of Greenhouse Gases. Retrieved March 13, 2016, from http://www3.epa.gov/climatechange/ghgemissions/gases/ch4.html .
- ↑ Schüring, J., Schulz, H. D., & Fischer, W. R. (Eds.). (2000). Redox: Fundamentals, processes, and applications. New York City, NY: Springer.
- ↑ Redox Chemistry Primer. (n.d.). Retrieved March 12, 2016, from http://www.kgs.ku.edu/Hydro/GWtutor/Plume_Busters/remediate_refs/redox_chemistry.htm .
- ↑ Madigan, M. T., Martinko, J. M., & Parker, J. (2003). Brock biology of microorganisms (10th ed.). Upper Saddle River, NJ: Prentice Hall/Pearson Education.
- ↑ 6.0 6.1 6.2 6.3 6.4 Silvia, D.M., et al. 2005. Principles and Applications of Soil Microbiology. 2nd ed. Pearson Prentice Hall, New Jersey.
- ↑ Occupational Safety & Health Administration. (n.d.). Safety and Health Topics | Hydrogen Sulfide. Retrieved February 23, 2016, from https://www.osha.gov/SLTC/hydrogensulfide/index.html .
- ↑ 8.0 8.1 Richardson, J. L., & Vepraskas, M. J. (2001). Wetland soils: Genesis, hydrology, landscapes, and classification. Boca Raton, FL: Lewis.
- ↑ Greenway H, William A, Timothy DC. (2006). Conditions leading to high CO2 (>5 kPa) in waterlogged-flooded soils and possible effects on root growth and metabolism. Annals of Botany, 98, 9–32. Retrieved March 10, 2016 from http://www.ncbi.nlm.nih.gov/pmc/articles/PMC3291891/ .
- ↑ De-Campos, A. B., Mamedov, A. I., & Huang, C. (2009). Short-Term Reducing Conditions Decrease Soil Aggregation. Soil Science Society of America Journal, 73(2), 550. Retrieved February 23, 2016, from https://dl.sciencesocieties.org/publications/sssaj/abstracts/73/2/550 .
- ↑ Scientific American. (2013, September 19). Soil Science: Make a Winogradsky Column. Retrieved from http://www.scientificamerican.com/article/bring-science-home-soil-column/
- ↑ Kirk, G. J. (2004). The biogeochemistry of submerged soils. Chichester: Wiley.
- ↑ Wenk, H., & Bulakh, A. G. (2004). Minerals: Their constitution and origin. Cambridge: Cambridge University Press.
- ↑ Minamikawa, K., & Sakai, N. (2005). The effect of water management based on soil redox potential on methane emission from two kinds of paddy soils in Japan. Agriculture, Ecosystems & Environment, 107(4), 397-407. Retrieved from http://www.sciencedirect.com/science/article/pii/S0167880904002208 .
(1) Lecture 5 of Kate Scow. 2008. Microbial Metabolism. Unpublished, University of California, Davis.
(2) Schlesinger, W.H. 1997. Biogeochemistry: An Analysis of Global Change. 2nd ed. Elsevier Academic Press, Amsterdam.
(4) J. Kotz, P. Treichel, G. Weaver. 2006. Chemistry and Chemical Reactivity sixth edition.
(6) Flood Duration Effects on Soybean Growth and Yield http://agron.scijournals.org/cgi/content/abstract/81/4/631
(10) MacFarlane, GT and Herbert, RA.,(1982) Nitrate dissimilation by Vibrio spp. isolated from estuarine sediments., Microbiology [J. GEN. MICROBIOL.]. Vol. 128, no. 10, pp. 2379-2383.
(11) S. M. Hasan and J. B. Hall, The physiological function of nitrate reduction inClostridium perfringens. J. Gen. Microbiol. 87 (1975), pp. 120–128
(12) Kuenen, J.G. and Robertson, L.A., 1988. Ecology of nitrification and denitrification. In: Cole, J.A. and Ferguson, S.J., Editors, 1988. The Nitrogen and Sulphur Cycles. 42nd Symposium of The Society for General Microbiology, University of Southampton, Cambridge University Press, Cambridge, pp. 161–218.
(13)Derek R. Lovley., 1993., Dissimilatory metal reduction. Annu Rev Microbiol. Vol 47 pp: 263-90.
(14) Gotoh, S and Patrick, W HJr 1974 Transformations of iron in waterlogged soils as influenced by redox potential and pH. Soil Sci. Soc. Am. Proc. 38, 66–71.
(15) [Schwertmann U & Taylor RM (1977) Iron oxides. Chap 5 In: Dixon JB & Weed SB (Eds) Minerals in Soil Environments (pp 145–180). Soil Sci. Soc. AM:; Madison, WI]
(19) Wenk H.R and Bulakh A ., 2004: Minerals. Their constitution and origin. Cambridge University Press.
(21) Matthews, E. and I. Fung. 1987. Methane Emission from Natural Wetlands: Global Distribution, Area, and Environmental Characteristics of Sources. Global Biogeochemical Cycles 1: 61-86.
See #Current Research section for a list of journals for additional information on the topic of flooded soils.
Edited by students of Kate Scow