Carbon cycle
Introduction
Soil stores at least three times as much carbon (in soil organic matter or SOM) as is found in either the atmosphere or in living plants (Fischlin, A, et al, 2007). A thorough understanding of the soil carbon cycle is essential to understanding the global carbon cycle, and “to the stewardship of ecosystem services provided by soils, such as fertility, water quality, resistant to erosion and climate mitigation through reduced feedbacks to climate changes(Schmidt, et al, 2011).” Atmospheric carbon, usually CO2, is brought into the biosphere by plants, where the carbon is fixed into various organic compounds. Soil microbes use the various components in plant residue as a source of energy and carbon for biosynthesis. This process is referred as decomposition. The easily degradable fraction of plant residue is turned into CO2 and microbial biomass quickly. As time goes, the intermediate fraction is also turned into CO2 and microbial biomass. The decomposition of the resistant fraction can take years, decades, or even centuries. All carbon compounds are eventually turned back into CO2, and released into atmosphere through microbial respiration and other processes. While complex carbon compounds are broken down into their constituents, new SOM is forming in the soil. Recent research has confirmed the importance of microbes in the formation of SOM. Microbes are the eye of the needle---carbon has to be filtered through for SOM to be formed.
The Carbon Cycle
Carbon Fixation
An important step of the carbon cycle is the fixation of atmospheric CO2 and its subsequent assimilation into organic molecules. Organisms that can convert CO2 into organic molecules are called autotrophs, and include plants, algae, some bacteria, and some archaea. Some bacteria specifically involved in carbon fixation are Cyanobacteria (such as Chroococcus, Nostoc, and Spirulina) and some species of Aquifex,such as Aquifex aeolicus. Cyanobacteria in the soil are also important Nitrogen fixers in the Nitrogen cycle including GHG.
Decomposition of Organic Matter and the Microbes Involved
Decomposition of organic residue is one of the major functions of microorganisms in the soil. The soil microorganisms utilize the residue components for energy and carbon source to synthesize the new cells. The organic materials added to soil are primarily plant residues from either cultivated crops or native vegetation, which are the source of energy stored in the carbon compounds. The crop agricultural ecosystems primarily include the combination of leaf, stem, and root tissues remaining after harvest. On the other hand, the residues that undergo decomposition in the natural ecosystems are derived from native prairie and forest vegetation. There are two types of plant residue that assimilate into the soil. One is above ground residue, which is derived from falling debris and other plant that are above the soil surface. The second type is deposited from root systems that complete their life cycle throughout the course of a particular season. These residue with the root exudates supply the substrate carbon input into the soil. The decay process of above-ground residue begin and initiate due to different agricultural management systems and practices. A portion of these substrate goes into microbial mass as it is used by the soil microorganisms for reproduction and metabolic activity and a larger part of it is lost as carbon dioxide due to the energy yielding metabolic processes of the microbial community. In the current study, Francesca Cotrufo emphasized that not all the litter Carbon loss are mineralized to carbon dioxide, but a part of these Carbon loss is transferred to mineral soil, where it contributes to the formation of soil organic matter through the two main pathways, dissolved organic matter-microbial path and physical transfer path (hyperlink to SOM path-reference M. Francesca Cotrufo).
Chemical Compounds
The composition of the crop residues added and decaying to soil are varied. Plant residues are comprised of many complex polymers such as lignin and cellulose and contains water-soluble organic compounds such as proteins, carbohydrates, and organic acids for the vast majority of microorganism in soil.
Proteins
Proteins are one of the most important components of plant residue added to soil. Protein is a polymers of amino acids linked by peptide bonds. A variety of microorganisms in soil can produce proteolytic enzymes such as protease and peptidase, which can break the peptide bond of the protein and hydrolyze it into individual amino acids. These amino acid monomers can then be utilized by microbes for either catabolism or for the synthesis of new essential proteins.(textbook)
Carbohydrates
From 5-25% of soil organic matter is combined in the form of carbohydrates, which include simple sugars, cellulose, and hemicellulose. These carbohydrates are rapidly degraded by varied microorganisms in the soil including bacteria, archaea, actinomycetes, and fungi. During degradation soil microorganisms synthesize extracellular polysaccharides. These polysaccharides bind soil into water stable aggregates so that the aggregates are more permeable to water and air. Polysaccharides also affect the cation exchange capacity of soil and act as an energy source for other microorganisms. Heterotrophic microbes can easily metabolize simple sugars. Plants link glucose molecules (and other sugar monomers) into long chains to produce polymers such as cellulose, which requires more specialized organisms to degrade. For example: Starch is made of amylose (alpha 1,4 bonded glucose) and amylopectin (alpha 1,6 bonded glucose), and is relatively easy for most organisms to degrade. Cellulose, however, is a polysaccharide of glucose connected with beta 1,4 bonds, and is more difficult to degrade. No animal can degrade cellulose, so bacteria can frequently be found in mutualistic relationships with detritivores: the bacteria degrade the cellulose enough that the animal is able to digest it.
Cellulose
Cellulose is the most abundant carbon input in soil. It’s a structural polysaccharide that is made of 1400 to 10000 glucose units. In order to access the glucose monomer, the cellulose must be cleaved by extracellular enzymes. These pieces are then transported into the cell for energy generation (catabolism) or production of biomass (anabolism). Cellulose is used by a diverse group of soil organisms including fungi such as Penicillium and Aspergillus and bacteria such as Streptomyces and Pseudomonas. Fungi and bacteria are important participants in the extracellular cleavage of cellulose [2].
Hemicellulose
Hemicellulose is the next most common carbohydrate in plants. It is a branched polymer with varied sugar monomers (glucose, mannose, and galactose) and bonds. The decomposition of hemicellulose is similar to that of cellulose in that the initial depolymerization step takes place outside of the cell, and the sugars produced are then transported into the cell for catabolism or anabolism. Even though hemicellulose decomposition is much quicker than cellulose decomposition, cells will utilize simple sugars as substrates before hemicellulose [2].
Chitin
Chitin is a special compound which can be found in the integument of arthropods and the cell walls of fungi. Chitin is one of the cell-wall component of many common soil fungi (e.g., Aspergillus and Penicillium) as around 3% to 25% of these soil fungal biomass are chitin(textbook). Chitin is usually protected from degradation by the protein-chitin complex in its natural normal stage in soil. Due to this type of protection, chitin is an important component in soil organic matter formation. The polymer is not easily degraded and requires a variety of enzymes to do so. The dominant chitin degraders are the actinomycetes Streptomyces and Nocardia. Less important than actinomycetes, fungi such as Trichoderma and Verticillium and bacteria, such as Bacillus and Pseudomonas can also degrade chitin.(textbook)
Lignin
Lignin is the main component of wood in trees. Lignin has a varied, unique, and complicated chemical structure which contains many aromatics. Aromatics are part of the reason why lignin is more difficult to be decomposed. Even with strong acid treatment, the plant residues are not solubilized due to the complex ring structure.These aromatics can be released from the lignin structure by fungal enzymes such as peroxidases and oxidases. The enzymes utilize H2O2 and OH radicals to break the bonds in the lignin. Lignin is decomposed through groups of specialized fungi. Common types of fungi which depolymerize lignin are white rot (Phanerochaete chrysosporium), brown rot, and soft rot. Once the aromatics are released from the original lignin structure they are incorporated into the metabolic pathway as pyruvate, acetyl CoA, and into the TCA cycle. Lignin stabilization through mineral interactions has recently been hypothesized to be a methodological artifact (Hernes et al. 2013).
Fats & Waxes
More than 2% of soil organic matter is cultivated in the forms, fats and waxes. Unlike cellulose and sugar, fats and waxes are much harder for microorganisms to breakdown. They are decomposed very slowly into CO2, water and energy which can be used by microorganisms. (6) Very little investigation has been done so information regarding this is limited.
Soil Organic Matter
The carbon cycle in soil is a dynamic balance between photosynthesis, the respiration of decomposing organisms, and the stabilization of carbon. Soil stores at least three times as much carbon (in SOM) as is found in either the atmosphere or in living plants (R). The total global carbon stock in soil is about 2500 Pg C, including approximately 1550 Pg in organic carbon, and 950 in inorganic carbon, as shown in figure #. Soils act as a buffer against the increase of atmospheric CO2. There is growing interest that it is possible to remove a significant amount of CO2 from the atmosphere by sequestering carbon in soil. It is estimated that 16-30% atmospheric CO2 can be removed when SOM concentrations increase 5-15% in soil up to 2m depth (Baldock, 2007; Kell 2011). It is also believed that soil respiration rates could cause a positive feedback in global warming. There are more nutrient based, plant-oriented interests in SOM, however, it seems that concerns about the changing climate “have now overtaken these other justifications”(Sollins 2007).
Growing Body of New Insights on SOM
For a long time, the scientific community described SOM in terms of humic substances. With the help of advanced analytical techniques, a growing body of research indicates that the importance of the biotic and abiotic environments in soil outweighs that of the molecular structure of plant inputs and organic matter in the process of OM stabilization. Factors such as rate of decay, SOM pools, stability, or ‘recalcitrance’ are not sufficient to describe the “ecosystem property” (Schmidt, 2011) of SOM; rather, texture, mineralogy, water solubility, molecular size, functionalization, and perhaps most important, climate change conditions should be incorporated into our understanding (and modeling) of carbon.
History of Humic Substances
Historically soil chemists use alkali and acid extraction methods and observations of the extracted (or residual) functional group chemistry to describe the soil organic matter, or humus. (Schmidt, et al, 2011) When protons are added to the solubilized organic materials, the dark color solid that precipitates is called ‘humic acid’. ‘Fulvic acid’ is the organic matter that remains soluble after reacidification treatment. The organic matter that does not respond to the extraction treatment is called ‘humin’. Long-standing theory suggests that humic substances are comprised of large and complex macromolecules which are the most stable SOM. Recently, by direct high-resolution observations, people understand that only a small portion of total organic matter is represented by humic substances. Smaller and simpler molecular structures are observed, as shown in Fig.1. The formation of humic polymers is not necessary related with humus formation in soils. A comprehensive review of traditional approach and critique of ‘humification’ model is conducted in the paper (Lehmann and Kleber, 2015). Biopolymer degradation and abiotic condensation (Frimmel & Christman, 1988) are the two general humification schemes developed on the knowledge of humus.
Decomposition and Molecular Structure
Historically SOM is thought to be made of stable and chemically unique compounds. The decomposition rate of plant residues largely depends on its biochemical composition, especially the C/N ratio and lignin content. Accordingly, people believe that the molecular structure of biomass and organic material controls the long-term decomposition rates in soil. Recent studies find that this is not true. Lignin and plant lipids (‘recalcitrants’) can turn over very rapidly. Moreover, sugar and other potential labile compounds have been shown to persist for decades---much longer than a couple weeks.
Role of Microbes in SOM Formation
“The role of microorganisms in SOM dynamics must be considered beyond the context of simple decomposition of fresh residues and extended to environment.” ---Jessica Chiartas, respectful TA of SSC111
Figure. Miltner 2012
Microorganisms' Role
Soil microorganisms serve not only as decomposers, but also as important components of SOM. Although plant residue contributes most of the carbon to soil, a large proportion actually ‘filters’ through microbial biomass before being transformed into SOM. It is now widely accepted that the contributions of microbial biomass to SOM formation seem to be much higher than the 1-5% estimation. A recent study shows that the living microbial biomass and necromass (the microbial residue after cell death) together account for 80% of the soil organic C (Liang and Balser 2010) .Bacteria and fungi comprise > 90% of the microbial biomass in soil (Six et al, 2006).
Microbes also contribute to soil aggregate formation, and soil structure. For instance, fungi help build microaggregates by connecting soil particles with their hyphae and extracellular components. (Six et al, 2006).
The turnover of microbial biomass can be described by microbial substrate use efficiency (SUE). Factors that affect SUE include 1) substrate quality ( molecular weight, solubility, structural complexity, C:N ratio), and 2) the degradative efficacy of microbes R( microbial community composition and structure, protection of organic compounds, other physiochemical environment). SUE is also a measure of the amount of ATP released through catabolism vs. the formation of biomolecules through anabolic activity, which is controlled by stoichiometric, chemical, and physiological factors (Cotrufo et al 2013). Microbes and SUE, being the eye of the needle through which carbon moves in soil, are good indicators of SOM formation. However, SUE does not equate to SOM stabilization. Details will be discussed in next section.
Recently, the similar compositions between SOM spectra and microbial biomass are observed by NMR approach (Simpson et al 2007a). Technology to 1) track the fate of specific labelled organic compounds and 2) directly observe microbes under high resolution scopes have extended our ability to understand soil microbial activity and diversity. Nevertheless, the quantitative linkages to ecosystem function remains uncertain (68 and 69 from Schmidt 2011). Detailed information should be linked to“the rates and routes of SOM processing” (Schmidt 2011).
Carbon (OM) Stability---Metabolic, Structural, ‘Recalcitrant’, and Beyond
“Rather than describing organic matter by decay rate, pool, stability or level of ‘recalcitrance’—as if these were properties of the compounds themselves—organic matter should be described by quantifiable environmental characteristics governing stabilization, such as solubility, molecular size and functionalization.” ---Michael W.I. Schmidt
Historically, SOM has been grouped into active, intermediate, and passive pools based on stabilization mechanisms and turnover time (Sollin et al, 1996) . SOM stability is controlled by accessibility rather than recalcitrance (Dungait et al. 2012). Stabilization is the protection of OM from mineralization. Stabilization refers to processes and mechanisms that can prolong C turnover times in soil. Detailed SOM stabilization mechanisms based on this knowledge have been well developed and summarized (Lutzow et al, 2008). However, a few mechanisms have been challenged in recent years(Schmidt 2011, Lehmann 2015). Managements of the size and the turnover rate of soil C pools can mitigate global climate change. Carbon pools with a fast turnover rate (<1 year) is important for short term nutrients availability in soil. SOM pools with slower turnover rate (decades, or even centuries) is important for soil structure and are believed to be more sensitive to climate change. After moving through the ‘microbial filter’, the fate of SOM is heavily dependent on environment conditions and soil properties (pH, soil texture, and mineralogy).Generally speaking, SOM stabilization is controlled by interactions of SOM with the mineral soil matrix through 1) phyllosilicates; 2) polyvalent cations (e.g., Ca2+); 3) Fe-, Al-, Mn-oxides; 4) spatial occurrence/accessibility(Cotrufo, 2013).
The formation of SOM and the decomposition of residue are intimately associated (Sylvia).
Recent SOM formation models with different focuses
“This does not mean that compound chemistry is not important for decomposition rates, just that its influence depends on environmental factors.” ---Michael W.I. Schmidt should find one from another person DELETE?
Three fairly modern conceptual models of SOM formation are selected and presented in this section. No single model is perfect. Advanced technology and previous knowledge are always “the shoulders of giant” to help us see further. Thus, models in this section should not be evaluated by comparing with each other; rather, they should be viewed collectively to help us to explore the world of soil carbon, where many things remain uncertain. SOM as Ecosystem property
Figure. Schmidt et al, 2011
From a respectful article, this model suggests that carbon stability should be viewed as an ecosystem property. The influence of compound chemistry largely depends on environment factors such as reactive soil mineral matrix, water solubility, soil redox state, pH and climate. Physical disconnection refers to the spatial inaccessibility between microbial decomposers and substrate, which causes SOM sequestration in aggregates and physical protection from decomposition. Sorption/desorption refers to the organo-mineral associations, mainly controlled by the amount and quality of silt and clay quality. The Fe-, Al-, and Mn-oxides,as clay coatings, interact with organic matters and physically protect organic matters. Thawing is expected to become more widespread due to climate change. It refers to the mineralization of previously stable SOM. Freezing, on the other hand, refers to the stabilization process owing to low temperatures. Microbial activity and products (necromass) affect the fate of SOM along the whole carbon cycle in soil.
Soil Continuum Model (SCM)
SCM is an evidence-base approach that focuses on the ability of decomposer organisms to access SOM and the protection of organic matter from decomposition. Molecular sizes of residues become smaller through the biotic transformation (microbial decomposer community), and the oxidation state of SOM increase. Consequently, the water solubility increases. At the same time, larger mineral surfaces and aggregates incorporation protect SOM against further decomposition. Abiotic transformations and estimate turnover time are also included.
Figure. Lehmann, 2015
Focusing on the Nexus of Plant Input and Decay
Recalcitrance is only important during early decomposition and in active surface soil (Lutzow, 2006)
Figure. Cotrufu. et al. 2015
Roles of SOM
Nutrient Cycling
Soil organic matter contributes 20% to 80% of the cation exchange capacity (CEC), which increase the ability of mineral soil to retain nutrients. SOM, itself, is a pool of nutrients for plant and microbes. SOM also enhances chelation (organic compounds forming complexes with cation like iron) and thus increase the bioavailability of trace elements to plants and other soil microbes.
Water Dynamics
Improves water infiltration. SOM take an important part in decreasing evaporation by enhancing the total water-holding capacity and strength of holding water in soil.
Strucutre
SOM contributes to improve soil structure and aggregation. Humic substances and polysaccharide produced by fungi and bacteria respectively stabilize and improve aggregation. As aggregation gets improved, the infiltration rate will be higher, which reduce runoff and soil erosion. SOM also helps to prevent compaction by decreasing bulk density and increasing percentage pore space.
Other Effects of SOM
Pesticides brehttps://microbewiki.kenyon.edu/index.php?title=Carbon_cycle&action=edit§ion=27ak down more quickly and can be "tied-up" by organic matter. Dark, bare soil may warm more quickly than light-colored soils, but heavy residue may slow warming and drying in spring. Plant residues and other organic material may support some diseases and pests, as well as predators and other beneficial organisms.
Factors Affecting SOM
A huge variety of factors can affect SOM. Countless organisms and chemical interactions affect its production and use. Understanding the many factors that contribute to the production, and state of SOM will give insight into everything from carbon sequestration and improving agricultural practices to the lives of microbial organisms.
- Quantity of Carbon: Carbon inputs are essential to soil as microbes are constantly respiring and releasing carbon from the soil. Low carbon inputs means there is less for microbes to metabolize and less carbon to be converted into organic matter there is more carbon available to be converted.
- Quality of Carbon: Easier to break down carbon sources will be converted to SOM faster. Though more complicate forms of carbon often produce a greater yield over time.
- Temperature: Colder weather in general means more soil organic matter. The cold slows microbial respiration so less carbon is metabolized.
- Moisture: Higher moisture leads to the same result. More water filled pores and less oxygen availability reduces microbial respiration and means less carbon released as carbon dioxide.
- Texture: High clay content means there is more surface area for carbon compounds to be attached to and more space for organomineral interaction. Furthermore, soil which is well aggregated can contain aggregates full of organic matter that are harder to reach for microbes to consume.
- Microbial Community: As shown in a lot of factors above, high microbial activity can lead to carbon being lost as a product of respiration. However, microbes are necessary as degraders of complex organic material, facilitating carbon mineralization, and are a large portion of organic matter itself as dead microbial biomass. Microbes have a specific C/N/P ratio and the more carbon there is the more carbon microbes will release as carbon dioxide. As a result, more proper carbon ratios more efficiently convert carbon into SOM.
- Disturbance: Soil disturbance such as tilling or erosion exposes protected pocket of organic matter and introduces oxygen reducing organic matter.
- Fire: Black carbon, carbon that has been burned and turned into charcoal, is integrated into soils by wildfires or human burning. This black carbon is being increasingly recognized as an important factor in SOM formation and retention. The charcoal particles have high surface area and surface charges. These factors give black carbon soils higher cation exchange capacities (3). A high charge means black carbon is very easily incorporated into SOM through mineralization.
Agriculture
Successful farming requires good, healthy soil. Good agricultural soil means lots of soil carbon and organic matter. The very nature of farming, growing and removing plants from soil again and again, removes a certain amount of carbon and other resources from the soil. However, a lot of the conventional practices around farming serve to damage soil and alter the carbon cycle far more than necessary. Many modern sustainable farming methods have been shown to greatly increase soil health. There are two different ways of approaching the problem, decreasing the amount of carbon lost and increasing the amount of carbon input.
Figure by Schurr, PhD. 2003
Reducing Carbon Losses
Carbon being lost from an agricultural system because of crop harvesting is unavoidable. The damage to soil can be reduced by growing less carbon intensive crops. Crops like corn return much of the plant carbon to the soil after a harvest from the husks and stalks of the plant. Another route by which carbon is lost from agricultural systems is via microbial respiration. Conventional farming greatly enhances these losses through tillage.
Tillage: Tillage is the practice of mixing and aerating soil by breaking it apart and turning it. Tilling practices increase oxygen availability to microbes and expose aggregate bound organic matter to microbes. As a result, high tillage increases microbial activity and soil respiration releasing previously soil bound carbon as carbon dioxide into the atmosphere. Tilling also damages soil structure, increasing erosion and removing yet more valuable organic carbon from fields. The increased attention on sustainable farming practices over the past decades has led to an increase in “no till” farming. In no till and reduced till systems levels of soil organic carbon, microbial biomass, and mineralizable nitrogen are significantly higher. In fact, gains in SOC were 250 kg/ha/yr higher in minimal till than in conventional systems. (2) Soil structure also improves greatly under no till farming. A reduction in tilling from conventional to no or minimal till increases macro aggregation by 21% - 42%. (2) The chart below shows the difference in the stratification ratio, a measure of organic matter at the surface of the soil over organic matter a bit deeper. No till systems have far more surface organic matter which helps fend off erosion and better facilitates seedling growth and root growth (7).
Change in stratification ratio of soil organic carbon with time under different tillage systems in Spain (from Franzluebbers, 2013)
Increasing Carbon Inputs
Practices for increasing carbon inputs have been used in traditional farming for a long time. Native americans often farmed polycultures, and crop rotations have been in use for hundreds of years. However, in modern conventional farming, where convenience of harvesting has outstripped the benefits of these techniques, there are very few carbon inputs to agricultural systems. While harder to implement in large scale farming these techniques are very important in practicing a sustainable model of agriculture. Monocultures, Crop Rotations, Polycultures, and Cover Crops: Monocultures, the system of growing a single crop continuously in the same soil, are common because of the simplicity of dealing with a single crop. However monoculture systems lead to lowered carbon, nitrogen, microbial biomass and diversity, and soil enzyme levels. One alternative to a monoculture is a crop rotation. Crop rotations are when alternating crops are grown in the soil. The benefits of crop rotation depend on what crops are rotated. Crop rotations shown to be especially beneficial to SOC levels include legumes and sweet clover. Both of these are often used as cover crops. Cover crops are, instead of being harvested, either tilled into, or laid on top of the soil. Cover cropping provides an annual boon of plant residue to the soil, massively increasing carbon in soil. Another system, less commonly used is that of a polyculture. A polyculture is a system in which multiple crops are grown at the same time. The diversity of crops allows for more diverse microbial communities and a greater variety of plant residue in the soil.
- Carbon Sequestration: The techniques that increase the amount of carbon in soil, no till with high carbon inputs, not only improve soil longevity in farming but also cause carbon sequestration. Carbon sequestration is the process by which carbon is taken from the atmosphere and stored long term, in this case as soil organic carbon. Switching from a conventional to a no till, organic system can sequester 22 gC/m2/yr. (2) There are movements all over the world to implement conservation farming methods. The sequestration of carbon in soil would not only increase the sustainability of farms, but also help reduce the carbon in the atmosphere, combating the buildup of greenhouse gases in the atmosphere.
- Recent Research: Recent research into conserving soil organic matter in agricultural systems suggests that minimizing tillage and introducing plant residues isn’t what ultimately conserves more organic matter. Microbial biomass is what ultimately creates organic matter. Results drawn by Kallenbach, Grandy, Frey, and Diefendorf (1) indicate that microbial growth rates and carbon use efficiency are associated with more conversion of carbon into microbial biomass and mineralized soil organic carbon. Enhancing the transformation of plant carbon to microbial biomass is a developing, exciting area of research that could lead to more effective agricultural management. Microbes, by breaking down organic molecules and facilitating the mineralization of carbon, are essential in storing carbon in soil for carbon sequestration.
Case Study
Permafrost Thaw Permafrost is a subsurface soil layer that remains frozen throughout the year. When the permafrost is thawed or unfrozen, it can either increase greenhouse gases emission or sequester more carbon in the carbon cycle. According to “Permafrost Thaw and Carbon Balance”, more than 50% of global terrestrial C is already stored in permafrost regions as soil organic matter. Carbon is naturally photosynthesized/uptaken by plants into soil ecosystem and respired in the form of CO2 back into atmosphere by plants, animals and microbes (bacteria). A carbon sink occurs when the uptake of CO2 by plants is greater than the emission of CO2 into the atmosphere by the process of respiration. A carbon source occurs when the atmosphere’s carbon content from respiring microbes and plants is greater than the uptake of CO2 by plants. As reported from “Permafrost Thaw and Carbon Balance”, permafrost thaw associated with climate warming can change the ecosystem carbon balance to a sink or a source (4). According to United Nations Environment Program, “[Thawing] of the Arctic permafrost is a “wild card” that could dramatically worsen global warming by releasing massive amounts of greenhouse gases…” (4). The reason global warming is associated with permafrost thaw is because, depending on the amount of permafrost thawed, it can stimulate great amounts of microbial decomposition of soil organic matter. This stimulation of decomposition will decrease the C content stored in soil by releasing more CO2 into the atmosphere. The CO2 that is released into atmosphere contributes to the cycle of greenhouse gases involved in climate change. In an experiment conducted by researchers from the University of Florida, C balance was intensively measured during the growing seasons in three separate conditions (minimally thawed permafrost, moderate thawed permafrost, extensive thawed permafrost). In the Figure below, the results of the study recorded accounted for both the growing and non-growing seasons. Researchers found that during non-growing seasons and minimal permafrost conditions, plants do not uptake C from the atmosphere but rather microorganisms release CO2 into the atmosphere indicated by the white bar in the Figure. In moderately permafrost thawed, C uptake exceeded C emission because of stimulation in decomposition which signified a carbon sink occurrence. Lastly, extensive permafrost thawed observed a C emission that exceeded C uptake which signified a carbon source occurrence that released large amounts of greenhouse gases to the atmosphere. Based on the measurements, Schuur concluded that “the net release of C to the atmosphere in landscapes where there is advanced permafrost thaw adds to the existing problem of increasing greenhouse gases in the atmosphere. This C release from permafrost thaw may create a dramatic feedback that accelerates climate change” (4)
Permafrost Thaw and Carbon Balance
Figure by Schuur. PhD, 2013
Drainage of Wetlands
Drainage of wetlands could either increase or decrease methane production but will certainly lose massive amounts of organic carbon sequestered in the soil from the carbon cycle. According to “The Role of Wetlands in the Carbon Cycle”, wetlands operate “six to nine percent of the Earth’s surface and contain about 35 percent of global terrestrial carbon.” (5). Wetlands are capable of high productivity meaning that they have a high capacity to sequester and store carbon. Wetlands sequester and store carbon into biomass and organic matter by photosynthesizing CO2 from the atmosphere. The wetland carbon cycle is displayed in the Figure below. Like permafrost thaw, seasons can affect wetlands by turning it into either a carbon sink or carbon source. As reported by the Department of Sustainability, Environment, Water, Population and Communities, wetlands are subjected to seasonal “waterlogging” conditions that induce anaerobic conditions (5). When the wetlands are subjected to inundated/anaerobic conditions, the emission of greenhouse gases (N2O and CH4) and carbon or methane sinks are created. It is because wetlands naturally store significant amounts of carbon, that during drainage conditions wetlands emit a significant amount of greenhouse gases. However, it is also noted that these drainage of channels also contribute to emission of methane to atmosphere. These “so-called” channels are hydrological connections that exist between watercourses and designated pathways to saturate wetlands with carbon and nutrients. During aerobic conditions, soil levels are oxygenated and methane production is decreased. Climate Change on Wetland Carbon Cycles As predicted by the Department of Sustainability, Environment, Water, Population and Communities, climate can play a part in the methane emission and carbon storage in wetland soils. Natural disturbances such as drainage of wetland soils will oxidize/aerate soils which decreases methane production which lead to large net losses of sediment organic carbon. In addition, depending on the climate change, wetland carbon cycle can altered in the following number of ways:
- Warmer climates will accelerate the rate of CO2 and methane production from wetland soils
- Wetter climates will increase wetland surface areas and promote carbon sequestration and increased primary production, but may increase methane emissions.
- Drier climates will increase the oxidation of carbon stores but reduce methane emissions.
Policies and Programs
A number of policies and outreach programs have been place to provide a positive feedback towards degradation of wetlands and emission of greenhouse gases by offsetting carbon sequestration and sources.. One of such policy called the Carbon Farming Initiative is currently carried out by the Department of Climate Change and Energy Efficiency. The policy is to implement change within the farmers’ lands. By storing excess greenhouse gas emission from wetlands into their lands, the farmers are able to earn carbon credits. Other organizations such as Regional Natural Resource Management Planning for Climate Change Fund and Biodiversity Fund are implementing policies to maintain healthy levels of greenhouse gas emission in wetlands.
Wetland Carbon Cycle Figure by Department of Sustainability, Environment, Water, Population and Communities, 2012
Atmospheric Changes Relating to the Carbon Cycle
Global Warming
Global warming is a result of a greenhouse effect caused by the accumulation of greenhouse gases in the atmosphere. The earth is heated by solar radiation that penetrates the atmosphere and is absorbed by the earth. Some of the enegy is reflected back into the atmosphere. Greenhouse gases prevent the escape of this solar energy, causing increased heating of the earth, a phenomenon known as global warming. The major greenhouse gases are water vapour, nitrous oxide (N2O), carbon dioxide (CO2), methane (CH4), and ozone (O3). Soil microorganisms play a role in the generation of each of these gases [3].
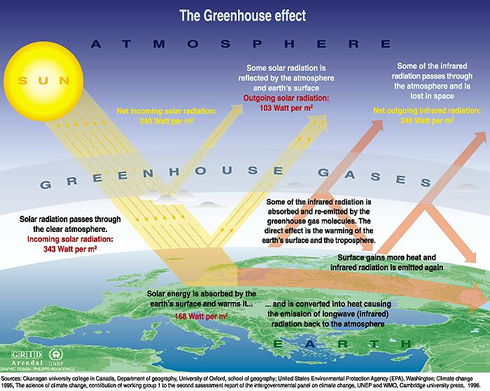
Water Vapour
Water vapour accounts 36% to 66% of the greenhouse effect. The water vapor content of the atmosphere is expected to increase in response to an increase in temperatures. "The increased water vapor in turn leads to an increase in the greenhouse effect and thus a further increase in temperature; the increase in temperature leads to still further increase in atmospheric water vapor; and the feedback cycle continues until equilibrium is reached. Thus water vapor acts as a positive feedback to the driving force provided by human-released greenhouse gases such as CO2" [5][8].
Nitrous Oxide
Microorganisms produce N2O by performing aerobic nitrification and anaerobic denitrification. Nitrification is the conversion of ammonia to nitrate. Nitrobacter hamburgensis and Nitrosococcus oceani are examples of nitrifying bacteria. Denitrification is the reduction of nitrate to nitrogen gas, with N2O as a side product. Pseudomonas and Flavobacterium are examples of denitrifying bacteria [2]. For more information on nitrogen transformation, see the Nitrogen cycle including GHG.
Carbon Dioxide
Microorganisms produce CO2 through the decomposition of organic matter. The balance between microbial repiration of CO2 and plant fixation of CO2 is important when considering microbial contribution to the greenhouse effect [2].
Methane
Methane is the end product of anaerobic respiration. This process is called methanogenesis. Microorganisms that produce methane through respiration are called Methanogens. There are two processes by which methanogens produce methane: CO2 reduction and acetate fermentation [2].
Methanotrophy is the process by which microorganisms, such as Methylobacterium, utilize methane as a carbon source. Carbon dioxide is a product of the reaction. These microorganisms are called methanotrophs [2].
Acid Rain
Nitrous oxides not only contribute to the greenhouse effect, but also to acid rain. Acid rain is corrosive, and increases the acidity of surface water and soils. Basic soils, including soils containing substantial amounts of calcium carbonate, can neutralize the acid rain. These soils are common in the Midwest, Great Plains, and many Western states [1].
Surface water becomes acidified when the buffering capacity of the water and soil is overwhelmed by the acid rain. The acid rain liberates aluminum from the soil. The aluminum then enters the water, posing a serious threat to aquatic organisms. Acidification of soils, and the associated release of aluminum, also poses a threat to soil microorganisms. Low pH can also release toxic metals such as lead, mercury, zinc, copper, cadmium, chromium, manganese, and vanadium from the soil. These mobilized ions inhibit the growth of soil bacteria and fungi [1].
The effects of acid rain also accelerate calcium carbonate (CaCO3) degradation. CaCO3 reacts with the acid to form CaO and CO2. The CO2 is released from the soil and combines with water vapor in the atmosphere to form more acid rain. This process, which continues until CaCO3 is completely consumed, is an example of a positive environmental feedback loop.
Current Research
Professor Joshua Schimel is studying the effect of global warming on the soil organic matter in the Arctic. The increasing temperature could result in increased evolution of CO2 to the atmosphere. On the other hand, plant growth could be fostered by the increased presence of nutrients from soil organic matter, making the Arctic a sink for CO2. The nature of the organic matter present in the Arctic soils will determine the type of environmental feedback. http://www.lifesci.ucsb.edu/eemb/faculty/schimel/research/research.html
The Urban Water Research Center at UC Irvine is conducting research on the effect of stengthened anaerobic degradation of manure on methane generation. http://www.uwrc.uci.edu/research-urban-water/UCI-UWRC-Research-Areas.php
Recently the EPA released a report analyzing the existing data on the connections between UV radiation, global climate change, and biogeochemical cycling. The report found that increased UV radiation affected the cycling of nutrients such as carbon and nitrogen. UV radiation impacts root exudation and the properties of dead plant material that become incorporated into the soil. This impacts microorganisms, and in turn, biogeochemical cycling. http://www.ncbi.nlm.nih.gov/pubmed/17344963?ordinalpos=1&itool=EntrezSystem2.PEntrez.Pubmed.Pubmed_ResultsPanel.Pubmed_DiscoveryPanel.Pubmed_Discovery_RA
References
1."Acid Rain - Soil Interactions" Available from: http://www.elmhurst.edu/~chm/vchembook/196soil.html
2.Sylvia, D.M., Fuhrmann, J.J., Hartel, P.G.,& Zuberer, D.A. (2005).Principles and Applications of Soil Microbiology, Second Edition. Prentice Hall, Upper Saddle River, NJ, 346,359,497-501 and 285-287 pp.
3.http://www.blackwell-synergy.com/doi/abs/10.1046/j.1365-2486.1997.00100.x KEITH SMITH (1997) "The potential for feedback effects induced by global warming on emissions of nitrous oxide by soils" Global Change Biology 3 (4) , 327–338 doi:10.1046/j.1365-2486.1997.00100.x
4.http://www.lifestyleblock.co.nz/articles/organics/02_organic_soil_matter.htm
5.realclimate.org http://www.realclimate.org/index.php?p=142 "Water vapour: feedback or forcing?"
6.http://www.gfdl.noaa.gov/reference/bibliography/2006/ih0601.pdf Issac M. Held, Brian J. Soden. (2006) "Robust Responses of the Hydrological Cycle to Global Warming " Journal of Climate volume19,issue = 21,pages5686-5699
7.Erickson DJ 3rd, Paul ND, Sulzberger B, Zepp RG. "Interactive effects of solar UV radiation and climate change on biogeochemical cycling," Photochem Photobiol Sci. 2007 Mar;6(3):286-300. Epub 2007 Feb 6.PMID: 17344963 [PubMed - indexed for MEDLINE] http://www.ncbi.nlm.nih.gov/pubmed/17344963?ordinalpos=1&itool=EntrezSystem2.PEntrez.Pubmed.Pubmed_ResultsPanel.Pubmed_DiscoveryPanel.Pubmed_Discovery_RA
8. http://en.wikipedia.org/wiki/Greenhouse_gas
Edited by student of Kate Scow